Chapter 1: Air Compressors
Introduction
Air compressors of various designs are used widely throughout DOE facilities in numerous applications. Compressed air has numerous uses throughout a facility including the operation of equipment and portable tools. Three types of designs include reciprocating, rotary, and centrifugal air compressors.
Reciprocating Compressors
The reciprocating air compressor, illustrated in Figure 1, is the most common design employed today. The reciprocating compressor normally consists of the following elements.
a. The compressing element, consisting of air cylinders, heads and pistons, and air inlet and discharge valves.
b. A system of connecting rods, piston rods, crossheads, and a crankshaft and flywheel for transmitting the power developed by the driving unit to the air cylinder piston.
c. A self-contained lubricating system for bearings, gears, and cylinder walls, including a reservoir or sump for the lubricating oil, and a pump, or other means of delivering oil to the various parts. On some compressors a separate force-fed lubricator is installed to supply oil to the compressor cylinders.
d. A regulation or control system designed to maintain the pressure in the discharge line and air receiver (storage tank) within a predetermined range of pressure.
e. An unloading system, which operates in conjunction with the regulator, to reduce or eliminate the load put on the prime mover when starting the unit.
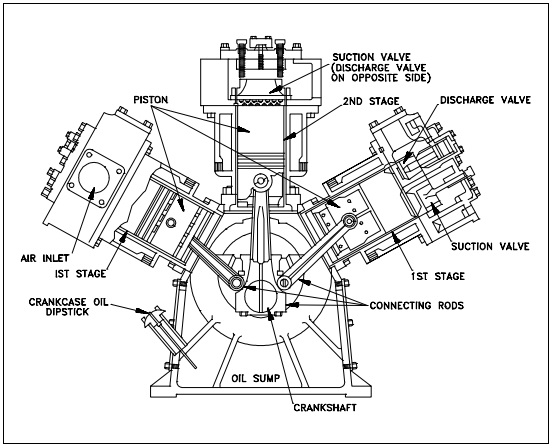
A section of a typical reciprocating single-stage, single-acting compressor cylinder is shown in Figure 2. Inlet and discharge valves are located in the clearance space and connected through ports in the cylinder head to the inlet and discharge connections.
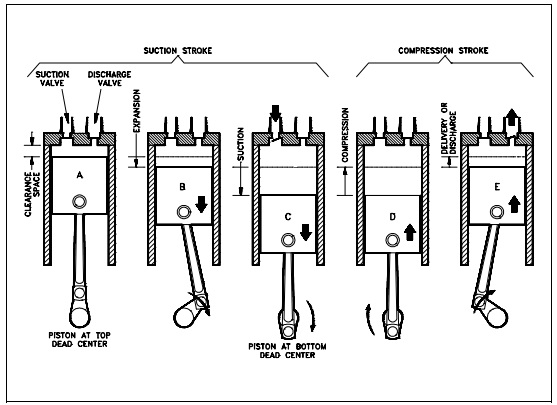
During the suction stroke the compressor piston starts its downward stroke and the air under pressure in the clearance space rapidly expands until the pressure falls below that on the opposite side of the inlet valve (Figures 2B and 2C). This difference in pressure causes the inlet valve to open into the cylinder until the piston reaches the bottom of its stroke (Figure 2C).
During the compression stroke the piston starts upward, compression begins, and at point D has reached the same pressure as the compressor intake. The spring-loaded inlet valve then closes. As the piston continues upward, air is compressed until the pressure in the cylinder becomes great enough to open the discharge valve against the pressure of the valve springs and the pressure of the discharge line (Figure 2E). From this point, to the end of the stroke (Figures 2E and 2A), the air compressed within the cylinder is discharged at practically constant pressure.
Rotary Compressors
The rotary compressor is adaptable to direct drive by induction motors or multicylinder gasoline or diesel engines. The units are compact, relatively inexpensive, and require a minimum of operating attention and maintenance. They occupy a fraction of the space and weight of a reciprocating machine of equivalent capacity. Rotary compressor units are classified into three general groups, slide vane-type, lobe-type, and liquid seal ring-type.
The rotary slide vane-type, as illustrated in Figure 3, has longitudinal vanes, sliding radially in a slotted rotor mounted eccentrically in a cylinder. The centrifugal force carries the sliding vanes against the cylindrical case with the vanes forming a number of individual longitudinal cells in the eccentric annulus between the case and rotor. The suction port is located where the longitudinal cells are largest. The size of each cell is reduced by the eccentricity of the rotor as the vanes approach the discharge port, thus compressing the air.
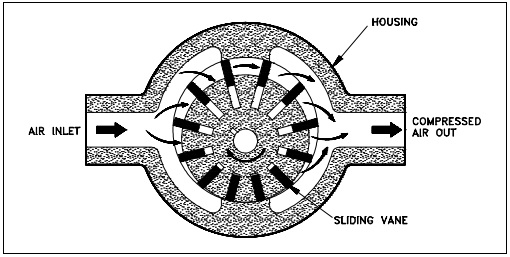
The rotary lobe-type, illustrated in Figure 4, features two mating lobe-type rotors mounted in a case. The lobes are gear driven at close clearance, but without metal-to-metal contact. The suction to the unit is located where the cavity made by the lobes is largest. As the lobes rotate, the cavity size is reduced, causing compression of the vapor within. The compression continues until the discharge port is reached, at which point the vapor exits the compressor at a higher pressure.

The rotary liquid seal ring-type, illustrated in Figure 5, features a forward inclined, open impeller, in an oblong cavity filled with liquid. As the impeller rotates, the centrifugal force causes the seal liquid to collect at the outer edge of the oblong cavity. Due to the oblong configuration of the compressor case, large longitudinal cells are created and reduced to smaller ones. The suction port is positioned where the longitudinal cells are the largest, and for the discharge port, where they are smallest, thus causing the vapor within the cell to compress as the rotor rotates. The rotary liquid seal compressor is frequently used in specialized applications for the compression of extremely corrosive and exothermic gasses and is commonly used in commercial nuclear plants as a means of establishing initial condenser vacuum.
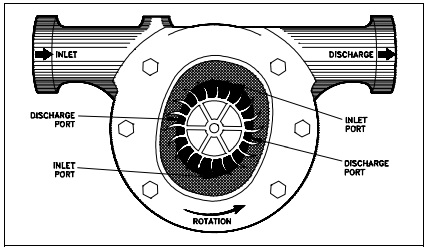
Centrifugal Compressors

The centrifugal compressor, originally built to handle only large volumes of low pressure gas and air (maximum of 40 psig), has been developed to enable it to move large volumes of gas with discharge pressures up to 3,500 psig. However, centrifugal compressors are now most frequently used for medium volume and medium pressure air delivery. One advantage of a centrifugal pump is the smooth discharge of the compressed air.
The centrifugal force utilized by the centrifugal compressor is the same force utilized by the centrifugal pump. The air particles enter the eye of the impeller, designated D in Figure 6. As the impeller rotates, air is thrown against the casing of the compressor. The air becomes compressed as more and more air is thrown out to the casing by the impeller blades. The air is pushed along the path designated A, B, and C in Figure 6. The pressure of the air is increased as it is pushed along this path. Note in Figure 6 that the impeller blades curve forward, which is opposite to the backward curve used in typical centrifugal liquid pumps. Centrifugal compressors can use a variety of blade orientation including both forward and backward curves as well as other designs.
There may be several stages to a centrifugal air compressor, as in the centrifugal pump, and the result would be the same; a higher pressure would be produced. The air compressor is used to create compressed or high pressure air for a variety of uses. Some of its uses are pneumatic control devices, pneumatic sensors, pneumatic valve operators, pneumatic motors, and starting air for diesel engines.
Compressor Coolers
The amount of moisture that air can hold is inversely proportional to the pressure of the air. As the pressure of the air increases, the amount of moisture that air can hold decreases. The amount of moisture that air can hold is also proportional to the temperature of the air. As the temperature of the air increases, the amount of moisture it can hold increases. However, the pressure change of compressed air is larger than the temperature change of the compressed air. This causes the moisture in the air to condense. Moisture in compressed air systems can cause serious damage. The condensed moisture can cause corrosion, water hammers, and freeze damage; therefore, it is important to avoid moisture in compressed air systems. Coolers are used to minimize the problems caused by heat and moisture in compressed air systems.
Coolers used on the discharge of a compressor are called aftercoolers. Their purpose is to remove the heat generated during the compression of the air. The decrease in temperature promotes the condensing of any moisture present in the compressed air. This moisture is collected in condensate traps that are either automatically or manually drained.
If the compressor is multi-staged, there may be an intercooler, which is usually located after the first stage discharge and before the second stage suction. The principle of the intercooler is the same as that of the aftercoolers. The result is drier, cooler, compressed air. The structure of a particular cooler depends on the pressure and volume of the air it cools. Figure 7 illustrates a typical compressor air cooler. Air coolers are used because drier compressed air helps prevent corrosion and cooler compressed air allows more air to be compressed for a set volume.
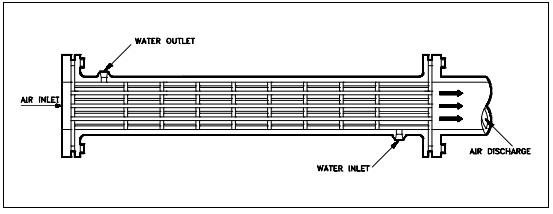
Hazards of Compressed Air
People often lack respect for the power in compressed air because air is so common and is often viewed as harmless. At sufficient pressures, compressed air can cause serious damage if handled incorrectly. To minimize the hazards of working with compressed air, all safety precautions should be followed closely.
Small leaks or breaks in the compressed air system can cause minute particles to be blown at extremely high speeds. Always wear safety glasses when working in the vicinity of any compressed air system. Safety goggles are recommended if contact lenses are worn.
Compressors can make an exceptional amount of noise while running. The noise of the compressor, in addition to the drain valves lifting, creates enough noise to require hearing protection. The area around compressors should normally be posted as a hearing protection zone.
Pressurized air can do the same type of damage as pressurized water. Treat all operations on compressed air systems with the same care taken on liquid systems. Closed valves should be slowly cracked open and both sides should be allowed to equalize prior to opening the valve further. Systems being opened for maintenance should always be depressurized before work begins.
Great care should be taken to keep contaminants from entering air systems. This is especially true for oil. Oil introduced in an air compressor can be compressed to the point where detonation takes place in a similar manner as that which occurs in a diesel engine. This detonation can cause equipment damage and personnel injury.
Chapter 2: Hydraulics
Introduction
Although any liquid can be used in a hydraulic system, some liquids have advantages over others. Oil is a liquid often preferred as the working fluid. Oil helps to lubricate the various sliding parts, prevents rust, and is readily available. For practical purposes, oil does not change its volume in the hydraulic system when the pressure is changed.
Pressure and Force
The foundation of modern hydraulic powered systems was established when a scientist named Blaise Pascal discovered that pressure in a fluid acts equally in all directions. This concept is known as Pascal’s Law. The application of Pascal’s Law requires the understanding of the relationship between force and pressure.
Force may be defined as a push or pull exerted against the total area of a surface. It is expressed in pounds. Pressure is the amount of force on a unit area of the surface. That is, pressure is the force acting upon one square inch of a surface.
The relationship between pressure and force is expressed mathematically.
F = P x A
where:
F = force in lbf
P = pressure in lbf/in.2 , (psi)
A = area in in.2
Hydraulic Operation
The operation of a typical hydraulic system is illustrated in Figure 8. Oil from a tank or reservoir flows through a pipe into a pump. Often a filter is provided on the pump suction to remove impurities from the oil. The pump, usually a gear-type, positive displacement pump, can be driven by an electric motor, air motor, gas or steam turbine, or an internal combustion engine. The pump increases the pressure of the oil. The actual pressure developed depends upon the design of the system.
Most hydraulic systems have some method of preventing overpressure. As seen in Figure 8, one method of pressure control involves returning hydraulic oil to the oil reservoir. The pressure control box shown on Figure 8 is usually a relief valve that provides a means of returning oil to the reservoir upon overpressurization.
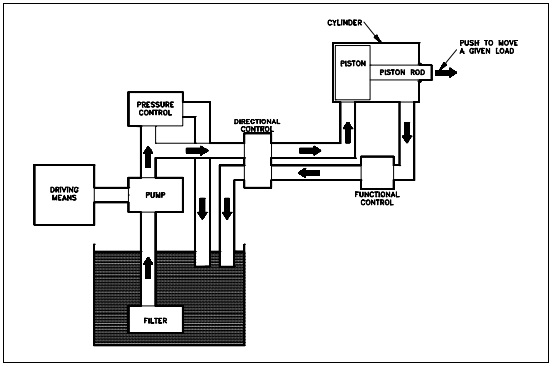
The high pressure oil flows through a control valve (directional control). The control valve Figure 8 Basic Hydraulic System changes the direction of oil flow, depending upon the desired direction of the load. In Figure 8 the load can be moved to the left or to the right by changing the side of the piston to which the oil pressure is applied. The oil that enters the cylinder applies pressure over the area of the piston, developing a force on the piston rod. The force on the piston rod enables the movement of a load or device. The oil from the other side of the piston returns to a reservoir or tank.
Hazards
The hazards and precautions listed in the previous chapter on air compressors are applicable to hydraulic systems as well, because most of the hazards are associated with high pressure conditions. Any use of a pressurized medium can be dangerous. Hydraulic systems carry all the hazards of pressurized systems and special hazards that are related directly to the composition of the fluid used.
When using oil as a fluid in a high pressure hydraulic system, the possibility of fire or an explosion exists. A severe fire hazard is generated when a break in the high pressure piping occurs and the oil is vaporized into the atmosphere. Extra precautions against fire should be practiced in these areas.
If oil is pressurized by compressed air, an explosive hazard exists if the high pressure air comes into contact with the oil, because it may create a diesel effect and subsequent explosion. A carefully followed preventive maintenance plan is the best precaution against explosion.
Chapter 3: Boilers and Cooling Towers
Introduction
The primary function of a boiler is to produce steam at a given pressure and temperature. To accomplish this, the boiler serves as a furnace where air is mixed with fuel in a controlled combustion process to release large quantities of heat. The pressure-tight construction of a boiler provides a means to absorb the heat from the combustion and transfer this heat to raise water to a temperature such that the steam produced is of sufficient temperature and quality (moisture content) for steam loads.
Boilers
Two distinct heat sources used for boilers are electric probes and burned fuel (oil, coal, etc.) This chapter will use fuel boilers to illustrate the typical design of boilers. Refer to Figure 9 during the following discussion.
The boiler has an enclosed space where the fuel combustion takes place, usually referred to as the furnace or combustion chamber. Air is supplied to combine with the fuel, resulting in combustion. The heat of combustion is absorbed by the water in the risers or circulating tubes. The density difference between hot and cold water is the driving force to circulate the water back to the steam drum. Eventually the water will absorb sufficient heat to produce steam.
Steam leaves the steam drum via a baffle, which causes any water droplets being carried by the steam to drop out and drain back to the steam drum. If superheated steam is required, the steam may then travel through a superheater. The hot combustion gasses from the furnace will heat the steam through the superheater’s thin tube walls. The steam then goes to the steam supply system and the various steam loads.
Some boilers have economizers to improve cycle efficiency by preheating inlet feedwater to the boiler. The economizer uses heat from the boiler exhaust gasses to raise the temperature of the inlet feedwater.

Fuel Boiler Components
Figure 9 illustrates a typical fuel boiler. Some of the components are explained below.
Steam drum – The steam drum separates the steam from the heated water. The water droplets fall to the bottom of the tank to be cycled again, and the steam leaves the drum and enters the steam system. Feedwater enters at the bottom of the drum to start the heating cycle.
Downcomers – Downcomers are the pipes in which the water from the steam drum travels in order to reach the bottom of the boiler where the water can enter the distribution headers.
Distribution headers – The distribution headers are large pipe headers that carry the water from the downcomers to the risers.
Risers – The piping or tubes that form the combustion chamber enclosure are called risers. Water and steam run through these to be heated. The term risers refers to the fact that the water flow direction is from the bottom to the top of the boiler. From the risers, the water and steam enter the steam drum and the cycle starts again.
Combustion chamber – Located at the bottom of a boiler, the combustion chamber is where and fuel mix and burn. It is lined with the risers.
Cooling Towers
Purpose
Before the development of cooling towers, rivers, lakes, and cooling ponds were required to supply cooling. Through the development of the mechanical draft cooling tower, as little as one square foot of area is needed for every 1000 square feet required for a cooling pond or lake. Cooling towers minimize the thermal pollution of the natural water heat sinks and allow the reuse of circulating water. An example of the manner in which a cooling tower can fit into a system is shown in Figure 10.

The cooling of the water in a cooling tower is accomplished by the direct contact of water and air. This cooling effect is provided primarily by an exchange of latent heat of vaporization resulting from evaporation of a small amount of water and by a transfer of sensible heat, which raises the temperature of the air. The heat transferred from the water to the air is dissipated to the atmosphere.
Induced Draft Cooling Towers
Induced draft cooling towers, illustrated in Figure 11, are constructed such that the incoming circulating water is dispersed throughout the cooling tower via a spray header. The spray is directed down over baffles that are designed to maximize the contact between water and air. The air is drawn through the baffled area by large circulating fans and causes the evaporation and the cooling of the water.
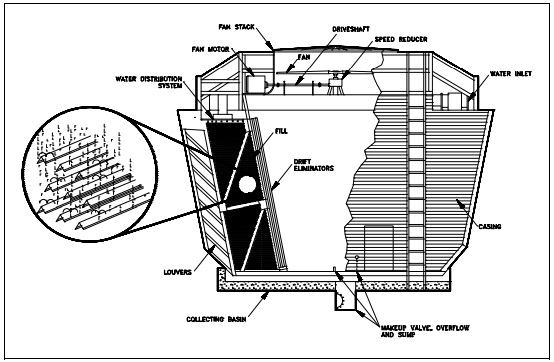
The nomenclature for induced draft cooling towers, including some items not illustrated in Figure 11 is summarized below.
Casing – The casing encloses the walls of the cooling tower, exclusive of fan deck and louvers.
Collecting basin – The collecting basin is a receptacle beneath the cooling tower for collecting the water cooled by the cooling tower. It can be made of concrete, wood, metal, or an alternative material. Certain necessary accessories are required such as sump, strainers, overflow, drain, and a makeup system.
Drift eliminators – The drift eliminators are parallel blades of PVC, wood, metal, or an alternative material arranged on the air discharge side of the fill to remove entrained water droplets from the leaving air stream.
Driver – The driver is a device that supplies power to turn the fan. It is usually an electric motor, but turbines and internal combustion engines are occasionally used.
Drive shaft – The drive shaft is a device, including couplings, which transmits power from the driver to the speed reducer.
Fan – The fan is a device used to induce air flow through the cooling tower.
Fan deck – The fan deck is a horizontal surface enclosing the top of the cooling tower above the plenum that serves as a working platform for inspection and maintenance.
Fan stack – The fan stack is a cylinder enclosing the fan, usually with an eased inlet and an expanding discharge for increased fan efficiency.
Fill – The fill is PVC, wood, metal, or an alternative material that provides extended water surface exposure for evaporative heat transfer.
Intake louvers – The intake louvers are an arrangement of horizontal blades at the air inlets that prevent escape of falling water while allowing the entry of air.
Makeup valve – The makeup valve is a valve that introduces fresh water into the collection basin to maintain the desired collecting basin water level.
Overflow – The overflow is a drain that prevents the collecting basin from overflowing.
Partition – The partition is a baffle within a multicell cooling tower that is used to prevent air and/or water flow between adjacent cells.
Plenum – The plenum is the internal cooling tower area between the drift eliminators and the fans.
Speed reducer – The speed reducer is a right-angle gear box that transmits power to the fan while reducing the driver speed to that required for optimal fan performance.
Sump – The sump is a depressed portion of the collecting basin from which cold water is drawn to be returned to the connected system. The sump usually contains strainer screens, antivortex devices, and a drain or cleanout connection.
Distribution system – The distribution system is that portion of a cooling tower that distributes water over the fill area. It usually consists of one or more flanged inlets, flow control valves, internal headers, distribution basins, spray branches, metering orifices, and other related components.
Forced Draft Cooling Towers
Forced draft cooling towers are very similar to induced draft cooling towers. The primary difference is that the air is blown in at the bottom of the tower and exits at the top. Forced draft cooling towers are the forerunner to induced draft cooling towers. Water distribution problems and recirculation difficulties discourage the use of forced draft cooling towers.
Natural Convection Cooling Towers
Natural convection cooling towers, illustrated in Figure 12, use the principle of convective flow to provide air circulation. As the air inside the tower is heated, it rises through the tower. This process draws more air in, creating a natural air flow to provide cooling of the water. The basin at the bottom of the tower is open to the atmosphere. The cooler, more dense air outside the tower will flow in at the bottom and contribute to the air circulation within the tower. The air circulation will be self perpetuating due to the density difference between the warmer air inside and the cooler air outside.
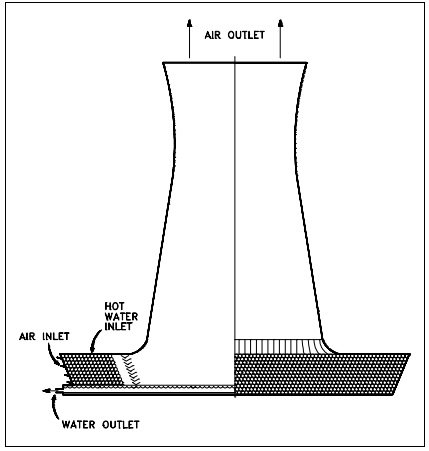
The incoming water is sprayed around the circumference of the tower and cascades to the bottom. The natural convection cooling towers are much larger than the forced draft cooling towers and cost much more to construct. Because of space considerations and cost, natural convection cooling towers are built less frequently than other types.
Chapter 4: Demineralizers
Purpose of Demineralizers
Dissolved impurities in power plant fluid systems generate corrosion problems and decrease efficiency due to fouled heat transfer surfaces. Demineralization of the water is one of the most practical and common methods available to remove these dissolved impurities.
In the plant, demineralizers (also called ion-exchangers) are used to hold ion exchange resins and transport water through them. Ion exchangers are generally classified into two groups: singlebed ion exchangers and mixed-bed ion exchangers.
Demineralizers
A demineralizer is basically a cylindrical tank with connections at the top for water inlet and resin addition, and connections at the bottom for the water outlet. The resin can usually be changed through a connection at the bottom of the tank. The resin beads are kept in the demineralizer by upper and lower retention elements, which are strainers with a mesh size smaller then the resin beads. The water to be purified enters the top at a set flow rate and flows down through the resin beads, where the flow path causes a physical filter effect as well as a chemical ion exchange.
Single-Bed Demineralizers
A single-bed demineralizer contains either cation or anion resin beads. In most cases, there are two, single-bed ion exchangers in series; the first is a cation bed and the second is an anion bed. Impurities in plant water are replaced with hydrogen ions in the cation bed and hydroxyl ions in the anion bed. The hydrogen ions and the hydroxyl ions then combine to form pure water. The Chemistry Handbook, Module 4, Principles of Water Treatment, addresses the chemistry of demineralizers in more detail.
Figure 13 illustrates a single-bed demineralizer. When in use, water flows in through the inlet to a distributor at the top of the tank. The water flows down through the resin bed and exits out through the outlet. A support screen at the bottom prevents the resin from being forced out of the demineralizer tank.

Single-Bed Regeneration
The regeneration of a single-bed ion exchanger is a three-step process. The first step is a backwash, in which water is pumped into the bottom of the ion exchanger and up through the resin. This fluffs the resin and washes out any entrained particles. The backwash water goes out through the normal inlet distributor piping at the top of the tank, but the valves are set to direct the stream to a drain so that the backwashed particles can be pumped to a container for waste disposal.
The second step is the actual regeneration step, which uses an acid solution for cation units and caustic solution for anion units. The concentrated acid or caustic is diluted to approximately 10% with water by opening the dilution water valve, and is then introduced through a distribution system immediately above the resin bed. The regenerating solution flows through the resin and out the bottom of the tank to the waste drain.
The final step is a rinsing process, which removes any excess regenerating solution. Water is pumped into the top of the tank, flows down through the resin bed and out at the bottom drain.
To return the ion exchanger to service, the drain valve is closed, the outlet valve is opened, and the ion exchanger is ready for service.
Single-bed demineralizers are usually regenerated “in place.” The resins are not pumped out to another location for regeneration. The regeneration process is the same for cation beds and for anion beds; only the regenerating solution is different. It is important to realize that if the ion exchanger has been exposed to radioactive materials, the backwash, regeneration, and rinse solutions may be highly radioactive and must be treated as a radioactive waste.
Mixed-Bed Demineralizer
A mixed-bed demineralizer is a demineralizer in which the cation and anion resin beads are mixed together. In effect, it is equivalent to a number of two-step demineralizers in series. In a mixed-bed demineralizer, more impurities are replaced by hydrogen and hydroxyl ions, and the water that is produced is extremely pure. The conductivity of this water can often be less than 0.06 micromhos per centimeter.
Mixed-Bed Regeneration
The mixed-bed demineralizer shown in Figure 14 is designed to be regenerated in place, but the process is more complicated than the regeneration of a single-bed ion exchanger. The steps in the regeneration are shown in Figure 14.
Figure 14a shows the mixed-bed ion exchanger in the operating, or on-line mode. Water enters through a distribution header at the top and exits through the line at the bottom of the vessel. Regeneration causes the effluent water to increase in electrical conductivity.
The first regeneration step is backwash, as shown in Figure 14b. As in a single-bed unit, backwash water enters the vessel at the bottom and exits through the top to a drain. In addition to washing out entrained particles, the backwash water in a mixed-bed unit must also separate the resins into cation and anion beds. The anion resin has a lower specific gravity than the cation resin; therefore, as the water flows through the bed, the lighter anion resin beads float upward to the top. Thus, the mixed-bed becomes a split bed. The separation line between the anion bed at the top and the cation bed at the bottom is called the resin interface. Some resins can be separated only when they are in the depleted state; other resins separate in either the depleted form or the regenerated form.
The actual regeneration step is shown in Figure 14c. Dilution water is mixed with caustic solution and introduced at the top of the vessel, just above the anion bed. At the same time, dilution water is mixed with acid and introduced at the bottom of the vessel, below the cation bed. The flow rate of the caustic solution down to the resin interface is the same as the flow rate of the acid solution up to the resin interface. Both solutions are removed at the interface and dumped to a drain.
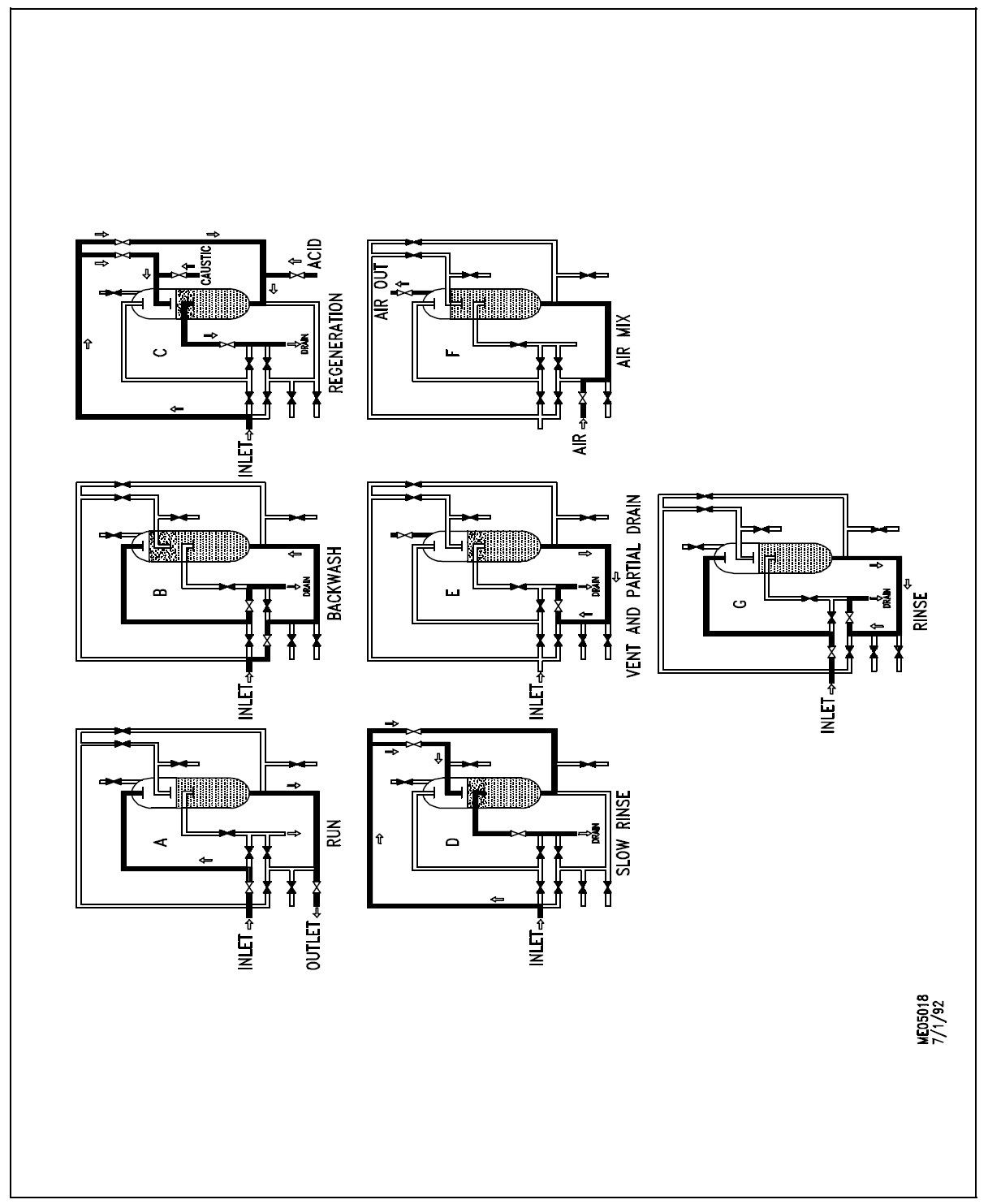
During the regeneration step, it is important to maintain the cation and anion resins at their proper volume. If this is not done, the resin interface will not occur at the proper place in the vessel, and some resin will be exposed to the wrong regenerating solution. It is also important to realize that if the ion exchanger has been involved with radioactive materials, both the backwash and the regenerating solutions may be highly radioactive and must be treated as liquid radioactive waste.
The next step is the slow rinse step, shown in Figure 14d, in which the flow of dilution water is continued, but the caustic and acid supplies are cut off. During this two-direction rinse, the last of the regenerating solutions are flushed out of the two beds and into the interface drain. Rinsing from two directions at equal flow rates keeps the caustic solution from flowing down into the cation resin and depleting it.
In the vent and partial drain step, illustrated in Figure 14e, the drain valve is opened, and some of the water is drained out of the vessel so that there will be space for the air that is needed to re-mix the resins. In the air mix step, (Figure 14f) air is usually supplied by a blower, which forces air in through the line entering the bottom of the ion exchanger. The air mixes the resin beads and then leaves through the vent in the top of the vessel. When the resin is mixed, it is dropped into position by slowly draining the water out of the interface drain while the air mix continues.
In the final rinse step, shown in Figure 14g, the air is turned off and the vessel is refilled with water that is pumped in through the top. The resin is rinsed by running water through the vessel from top to bottom and out the drain, until a low conductivity reading indicates that the ion exchanger is ready to return to service.
External Regeneration
Some mixed-bed demineralizers are designed to be regenerated externally, with the resins being removed from the vessel, regenerated, and then replaced. With this type of demineralizer, the first step is to sluice the mixed bed with water (sometimes assisted by air pressure) to a cation tank in a regeneration facility. The resins are backwashed in this tank to remove suspended solids and to separate the resins. The anion resins are then sluiced to an anion tank. The two batches of separated resins are regenerated by the same techniques used for single-bed ion exchangers. They are then sluiced into a holding tank where air is used to remix them. The mixed, regenerated, resins are then sluiced back to the demineralizer. External regeneration is typically used for groups of condensate demineralizers. Having one central regeneration facility reduces the complexity and cost of installing several demineralizers.
External regeneration also allows keeping a spare bed of resins in a holding tank. Then, when a demineralizer needs to be regenerated, it is out of service only for the time required to sluice out the depleted bed and sluice a fresh bed in from the holding tank. A central regeneration facility may also include an ultrasonic cleaner that can remove the tightly adherent coating of dirt or iron oxide that often forms on resin beads. This ultrasonic cleaning reduces the need for chemical regeneration.
Chapter 5: Pressurizers
Introduction
There are two types of pressurizers: static and dynamic. A static pressurizer is a partially filled tank with a required amount of gas pressure trapped in the void area. A dynamic pressurizer is a tank in which its saturated environment is controlled through use of heaters (to control temperature) and sprays (to control pressure).
This chapter focuses on the dynamic pressurizer. A dynamic pressurizer utilizes a controlled pressure containment to keep high temperature fluids from boiling, even when the system undergoes abnormal fluctuations.
Before discussing the purpose, construction, and operation of a pressurizer, some preliminary information about fluids will prove helpful.
The evaporation process is one in which a liquid is converted into a vapor at temperatures below the boiling point. All the molecules in the liquid are continuously in motion. The molecules that move most quickly possess the greatest amount of energy. This energy occasionally escapes from the surface of the liquid and moves into the atmosphere. When molecules move into the atmosphere, the molecules are in the gaseous, or vapor, state.Liquids at a high temperature have more molecules escaping to the vapor state, because the molecules can escape only at higher speeds. If the liquid is in a closed container, the space above the liquid becomes saturated with vapor molecules, although some of the molecules return to the liquid state as they slow down. The return of a vapor to a liquid state is called condensation. When the amount of molecules that condense is equal to the amount of molecules that evaporate, there is a dynamic equilibrium between the liquid and the vapor.
Pressure exerted on the surface of a liquid by a vapor is called vapor pressure. Vapor pressure increases with the temperature of the liquid until it reaches saturation pressure, at which time the liquid boils. When a liquid evaporates, it loses its most energetic molecules, and the average energy per molecule in the system is lowered. This causes a reduction in the temperature of the liquid.
Boiling is the activity observed in a liquid when it changes from the liquid phase to the vapor phase through the addition of heat. The term saturated liquid is used for a liquid that exists at its boiling point. Water at 212o°F and standard atmospheric pressure is an example of a saturated liquid.Saturated steam is steam at the same temperature and pressure as the water from which it was formed. It is water, in the form of a saturated liquid, to which the latent heat of vaporization has been added. When heat is added to a saturated steam that is not in contact with liquid, its temperature is increased and the steam is superheated. The temperature of superheated steam, expressed as degrees above saturation, is called degrees of superheat.
General Description
The pressurizer provides a point in the reactor system where liquid and vapor can be maintained in equilibrium under saturated conditions, for control purposes. Although designs differ from facility to facility, a typical pressurizer is designed for a maximum of about 2500 psi and 680°F.
Dynamic Pressurizers
A dynamic pressurizer serves to:
• maintain a system’s pressure above its saturation point,
• provide a means of controlling system fluid expansion and contraction,
• provide a means of controlling a system’s pressure, and
• provide a means of removing dissolved gasses from the system by venting the vapor space of the pressurizer.
Construction

A dynamic pressurizer is constructed from a tank equipped with a heat source such as electric heaters at its base, a source of cool water, and a spray nozzle. A spray nozzle is a device located in the top of the pressurizer that is used to atomize the incoming water.
A dynamic pressurizer must be connected in the system to allow a differential pressure to exist across it. The bottom connection, also called the surge line, is the lower of the two pressure lines. The top connection, referred to as the spray line, is the higher pressure line. Differential pressure is obtained by connecting the pressurizer to the suction and discharge sides of the pump servicing the particular system. Specifically, the surge (bottom connection) is connected to the pump’s suction side; the spray line (top connection) is connected to the pump’s discharge side. A basic pressurizer is illustrated in Figure 15.
The hemispherical top and bottom heads are usually constructed of carbon steel, with austenitic stainless steel cladding on all surfaces exposed to the reactor system water.
The pressurizer can be activated in two ways. Partially filling the pressurizer with system water is the first. After the water reaches a predetermined level, the heaters are engaged to increase water temperature. When the water reaches saturation temperature, it begins to boil. Boiling water fills the void above the water level, creating a saturated environment of water and steam. The other method involves filling the pressurizer completely, heating the water to the desired temperature, then partially draining the water and steam mixture to create a steam void at the top of the vessel.
Water temperature determines the amount of pressure developed in the steam space, and the greater the amount of time the heaters are engaged, the hotter the environment becomes. The hotter the environment, the greater the amount of pressure.
Installing a control valve in the spray line makes it possible to admit cooler water from the top of the pressurizer through the spray nozzle. Adding cooler water condenses the steam bubble, lowers the existing water temperature, and reduces the amount of system pressure.
Operation
The level of water within a pressurizer is directly dependant upon the temperature, and thus the density, of the water in the system to which the pressurizer is connected. An increase in system temperature causes the density of the water to decrease. This decreased density causes the water to expand, causing the level of water to increase in the vessel. The increased level of water in a pressurizer is referred to as an insurge. An insurge compresses the vapor space, which in turn causes the system pressure to rise. This results in slightly superheated steam in contact with the subcooled pressurizer liquid. The superheated steam transfers heat to the liquid and to the pressurizer walls. This re-establishes and maintains the saturated condition.
A decrease in system temperature causes the density to increase which causes the system water volume to contract. The contraction (drop) in pressurizer water level and increase in vapor space is referred to as an outsurge. The increase in vapor space causes the pressure to drop, flashing the heated water volume and creating more steam. The increased amount of steam re-establishes the saturated state. Flashing continues until the decrease in water level ceases and saturated conditions are restored at a somewhat lower pressure.
In each case, the final conditions place the pressurizer level at a new value. The system pressure remains at approximately its previous value, with relatively small pressure variations during the level change, provided that the level changes are not too extreme.
In actual application, relying on saturation to handle all variations in pressure is not practical. In conditions where the system water is surging into the pressurizer faster than the pressurizer can accommodate for example, additional control is obtained by activating the spray. This spray causes the steam to condense more rapidly, thereby controlling the magnitude of the pressure rise.
When a large outsurge occurs, the level can drop rapidly and the water cannot flash to steam fast enough. This results in a pressure drop. The installed heaters add energy to the water and cause it to flash to steam faster, thereby reducing the pressure drop. The heaters can also be left on to re-establish the original saturation temperature and pressure. In certain designs, pressurizer heaters are energized continuously to make up for heat losses to the environment.
The pressurizer’s heater and spray capabilities are designed to compensate for the expected surge volume. The surge volume is the volume that accommodates the expansion and contraction of the system, and is designed to be typical of normal pressurizer performance. Plant transients may result in larger than normal insurges and outsurges. When the surge volume is exceeded, the pressurizer may fail to maintain pressure within normal operating pressures.
Pressurizer operation, including spray and heater operation, is usually automatically controlled. Monitoring is required in the event the control features fail, because the effect on the system could be disastrous without operator action.
Chapter 6: Steam Traps
General Operation

In general, a steam trap consists of a Figure 16 Ball Float Steam Trap valve and a device or arrangement that causes the valve to open and close as necessary to drain the condensate from piping without allowing the escape of steam. Steam traps are installed at low points in the system or machinery to be drained. Some types of steam traps that are used in DOE facilities are described in this chapter.
Ball Float Steam Trap
A ball float steam trap is illustrated in Figure 16. The valve of this trap is connected to the float in such a way that the valve opens when the float rises. When the trap is in operation, the steam and any water that may be mixed with it flows into the float chamber. The water, being heavier than the steam, falls to the bottom of the trap, causing the water level to rise. As the water level rises, it lifts the float; thus lifting the valve plug and opening the valve. The condensate drains out and the float moves down to a lower position, closing the valve before the condensate level gets low enough to allow steam to escape. The condensate that passes out of the trap is returned to the feed system.
Bucket Steam Trap
A bucket steam trap is illustrated in Figure 17. As condensate enters the trap body, the bucket floats. The valve is connected to the bucket in such a way that the valve closes as the bucket rises. As condensate continues to flow into the trap body, the valve remains closed until the bucket is full. When the bucket is full, it sinks and thus opens the valve. The valve remains open until enough condensate has passed out to allow the bucket to float, and closing the valve.

Thermostatic Steam Traps
There are several kinds of thermostatic steam traps in use. In general, these traps are more compact and have fewer moving parts than most mechanical steam traps.
Bellows-Type Steam Trap
A bellows-type steam trap is illustrated in Figure 18. The operation of this trap is controlled by the expansion of the vapor of a volatile liquid, which is enclosed in a bellows-type element. Steam enters the trap body and heats the volatile liquid in the sealed bellows, causing expansion of the bellows.
The valve is attached to the bellows in such a way that the valve closes when the bellows expands. The valve remains closed, trapping steam in the valve body. As the steam cools and condenses, the bellows cools and contracts, thereby opening the valve and allowing the condensate to drain.
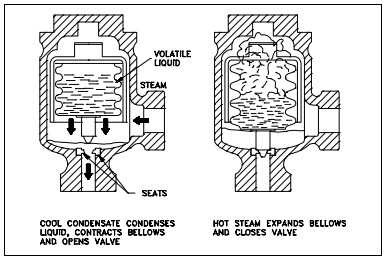
Impulse Steam Trap
Impulse steam traps, illustrated in Figure 19, pass steam and condensate through a strainer before entering the trap. A circular baffle keeps the entering steam and condensate from impinging on the cylinder or on the disk. The impulse type of steam trap is dependent on the principle that hot water under pressure tends to flash into steam when the pressure is reduced.
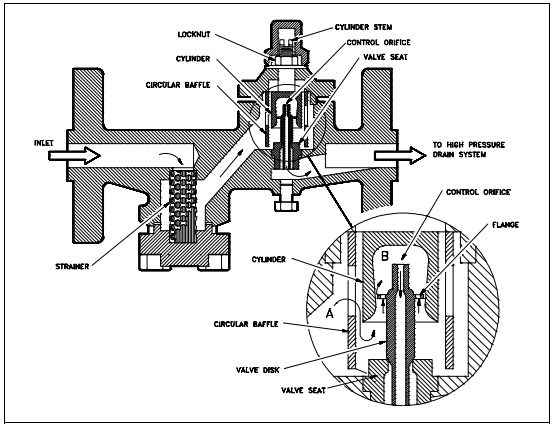
The only moving part in the steam trap is the disk. A flange near the top of the disk acts as a piston. As demonstrated in Figure 19, the working surface above the flange is larger than the working surface below the flange.
A control orifice runs through the disk from top to bottom, which is considerably smaller at the top than at the bottom. The bottom part of the disk extends through and beyond the orifice in the seat. The upper part of the disk (including the flange) is inside a cylinder. The cylinder tapers inward, so the amount of clearance between the flange and the cylinder varies according to the position of the valve. When the valve is open, the clearance is greater than when the valve is closed.
When the trap is first placed in service, pressure from the inlet (chamber A) acts against the underside of the flange and lifts the disk off the valve seat. Condensate is thus allowed to pass out through the orifice in the seat; and, at the same time, a small amount of condensate (called control flow) flows up past the flange and into chamber B. The control flow discharges through the control orifice, into the outlet side of the trap, and the pressure in chamber B remains lower than the pressure in chamber A.
As the line warms up, the temperature of the condensate flowing through the trap increases. The reverse taper of the cylinder varies the amount of flow around the flange until a balanced position is reached in which the total force exerted above the flange is equal to the total force exerted below the flange. It is important to note that there is still a pressure difference between chamber A and chamber B. The force is equalized because the effective area above the flange is larger than the effective area below the flange. The difference in working area is such that the valve maintains at an open, balanced, position when the pressure in chamber B is approximately 86% of the pressure in chamber A.
As the temperature of the condensate approaches its boiling point, some of the control flow going to chamber B flashes into steam as it enters the low pressure area. Because the steam has a much greater volume than the water from which it is generated, pressure builds up in the space above the flange (chamber B). When the pressure in this space is 86% of the inlet pressure (chamber A), the force exerted on the top of the flange pushes the entire disk downward and closes the valve. With the valve closed, the only flow through the trap is past the flange and through the control orifice. When the temperature of the condensate entering the trap drops slightly, condensate enters chamber B without flashing into steam. Pressure in chamber B is thus reduced to the point where the valve opens and allows condensate to flow through the orifice in the valve seat. The cycle is repeated continuously.
With a normal condensate load, the valve opens and closes at frequent intervals, discharging a small amount of condensate at each opening. With a heavy condensate load, the valve remains open and allows a continuous discharge of condensate.
Orifice-Type Steam Trap
Some industry facilities may use continuous-flow steam traps of the orifice type in some constant service steam systems, oil-heating steam systems, ventilation preheaters, and other systems or services in which condensate forms at a fairly constant rate. Orifice-type steam traps are not suitable for services in which the condensate formation is not continuous.
Although there are several variations of the orifice-type steam trap, each has one thing in common; it contains no moving parts. One or more restricted passageways or orifices allow condensate to trickle through, but do not allow steam to flow through. Some orifice-type steam traps have baffles in addition to orifices.
Chapter 7: Filters And Strainers
Introduction
Filtration is a process used to remove suspended solids from a solution. Other processes such as demineralization remove ions or dissolved ions. Different filters and strainers are used for different applications. In general, the filter passage must be small enough to catch the suspended solids but large enough that the system can operate at normal system pressures and flows. Filters and strainers are used throughout most DOE facilities. They are used in hydraulic systems, oil systems, cooling systems, liquid waste disposal, water purification, and reactor coolant systems.
Cartridge Filters
Figure 20 illustrates a typical multi-cartridge filter. The cartridges are cylinders and usually consist of a fiber yarn wound around a perforated metal core. The liquid being filtered is forced through the yarn, which is approximately 1/2 inch thick, and then through the perforations in the metal core to the filter outlet, which can be at either end. A cartridge filter may include several cartridges, the exact number depending on the liquid flow rate that must be handled.

In the filter assembly illustrated in Figure 21, the cartridges are held between plates so that the water must pass through the layer of yarn to reach the filter outlet. The type of yarn that is used depends on the application. Some of the fibers commonly used include resin-impregnated wool or cellulose, cotton-viscose, polypropylene, nylon, and glass. In some applications that involve high temperatures or pressures, porous metal cartridges are used. These cartridges are usually made of 316 stainless steel, but inconel, monel, and nickel are also used.
Depending on the fiber or metal that is used, Figure 21 Cartridge Filter cartridges are available that will filter out all particle matter down to a specified size. For example, a certain cartridge might be designed to remove all particles larger than 10 microns, one micron, or even 0.1 micron. (A micron is 10-3 millimeters.)

Cartridge filters have the advantage of being relatively inexpensive to install and operate. Instruments measure the differential pressure across these filters to let the operator know when a filter is plugged and must be replaced. When the cartridges are removed from radioactive systems, the radiation levels can be very high. For this reason, the cartridges may be withdrawn into a shielded cask for moving to a storage area or a solid waste processing area. When the porous metal cartridges become plugged, they can be cleaned ultrasonically and reused. When this is done, the cleaning solution becomes contaminated and must be processed as liquid radioactive waste.
Another type of cartridge filter is the wafer, or disk filter. In this filter, disks are stacked to form a cartridge and placed down over a central perforated pipe. Each disk is typically 1/8 inch to 1/4 inch thick and made of cellulose or asbestos fibers.
Liquid that enters the disk filter moves up around the outside of the stack of disks, is forced between the disks, travels through the perforations in the central pipe, and then leaves the filter. The filtering action takes place as the liquid is forced between the disks.
As with the smaller cartridges, if a disk filter is used to filter radioactive water, it may be very radioactive when it is removed, and must be handled very carefully. One way to remove a disk filter is by means of a crane, which lifts the filter out of its housing and moves it to a shielded container. The disposal problem is one of the major disadvantages of cartridge and diskcartridge filters.
Precoat Filters
A precoat filter eliminates the problem of physically handling radioactive materials, because the filter material (called the medium) can be installed and removed remotely. Inside the filter housing is a bundle of septums (vertical tubes, on which the filter medium is deposited). The septums in some filters are approximately 1 inch in diameter and 3 feet long and are usually made of perforated or porous metal (normally stainless steel). There may be several hundred of these septums in a filter. Septums in other filters are approximately 3 inches in diameter and 3 feet long and are made of porous stone or porous ceramic material. There are usually less than 100 of these larger septums in a filter.
The filtering medium fibers may be finely divided diatomite, perlite, asbestos, or cellulose. Diatomite, the least expensive medium, is used to filter liquid waste that will be discharged from the plant. Cellulose is generally used for processing water that will be returned to a reactor, because diatomite can allow silica leaching.
When a precoat filter is in use, water that enters the filter vessel passes through the filter medium that is deposited on the septums and then leaves through the outlet. Before the filter can be placed into operation, however, the filter medium must be installed; that is, the filter must be precoated.
The first step in precoating the filter is to close the inlet and outlet valves to the filter. The filter medium used is mixed with demineralized water in an external mixing tank to form a slurry, which is pumped through the filter. Some of the filter medium deposits on the septums and is held there by the pressure of water on the outside of the septums. At the beginning of the precoating process, some of the fibers of the filter medium pass through the septums, either because they are smaller than the openings or because they pass through lengthwise. Thus, there is still some filter medium in the water as it leaves the filter, so the slurry is recirculated again and again until the water is clear. Clear water indicates that all of the filter medium is deposited on the septums, and the filter is precoated.
One characteristic of the precoating process is that a very even layer of filter medium (approximately 1/8 inch thick) is deposited on the septums. This occurs because the circulating slurry follows the path of least resistance. When the coating at one point reaches a certain thickness, the slurry takes the fibers to another point, and this process continues until precoating is complete.
Because water pressure holds the filter in place, flow must be maintained through the recirculating loop to keep the medium from falling off. This is called a holding flow. As the inlet and outlet valves are opened for normal usage, called service flow, the holding flow is gradually cut off.
Backwashing Precoat Filters
After a filter has been precoated, it is put into service and kept on line until the pressure differential indicates that the filter medium is becoming plugged. When this occurs, the old filter medium is removed and the filter is precoated again. Filters are usually installed in pairs, so that one filter can remain in service while the other is undergoing the filter backwashing and precoating process.
Since water pressure helps to hold the filter medium against the septums, some of the old filter medium will fall off as soon as this pressure is removed. Backwashing is used to remove the filter medium that does not fall off. Backwashing is usually done in one of two ways. With some filters, demineralized water is pumped backwards through the center of the septums, and the filter medium coating is knocked off by the water as it comes out through the septums.
Most filters use a multi-step backwashing procedure. First, the inlet valve and the outlet valve are closed, and the drain valve and the top vent are opened to allow the water to drain. Then the drain valve and the vent are closed, and the inlet water valve is opened to raise the water level. The filter is equipped with a special high-domed top to trap and compress air. When the water inlet valve is closed and the drain valve is opened quickly, the compressed air forces water down through the center of the septums. This water knocks the filter medium off of the septums.
With both types of backwashing, the filter medium coating that is removed is sluiced out through a drain line to a filter sludge tank, where it is stored for further processing. The filter is then precoated again and put back into service.
With precoat filters, the type and quantity of filter medium is critical. If too little material or too coarse a material is used, some of the finely divided crud in the water may get into the openings of the septums. When the filter is backwashed, this crud is usually not removed. It continues to build up during subsequent use of the filter until the septums become so plugged that they have to be replaced.
If too much filter medium is used, the layer that builds up on the septums will bridge the area between the septums. When the filter is backwashed, these bridges are usually not removed. Therefore the bridging continues, and the filter runs become progressively shorter. Eventually, the filter must be opened and the filter medium must be removed manually.
Precoat filters are much more complicated than cartridge filters, and the equipment required is much more expensive to install and maintain. The major advantage of precoat filters is the remote operation, which eliminates the physical handling of highly radioactive filter cartridges.
Deep-Bed Filters

Deep-bed filters are usually found only in makeup water systems, where they are used to filter water after it has been treated in a clarifier. They are used to remove organic matter, chlorine, and very fine particulate matter.
A deep-bed filter is based on a support (decking), which is mounted a few inches above the bottom of the tank. The screen is perforated to allow water to flow through it. A coarse, aggregate layer of crushed rock or large lumps of charcoal is placed on top of the screen, and the deep bed itself (2 to 4 feet of granular anthracite or charcoal) is placed on top of the aggregate. The filter is sized so that there is 1 to 2 feet of “free board” above the deep bed.
When the filter is in service, raw water is pumped in through a pipe that feeds a distribution pipe above the deep bed. The water is filtered as it percolates down through the granules. (Charcoal granules will filter out organic matter, chlorine, and fine particulates, while anthracite granules remove only the particulates.) The water collects in the bottom of the tank, below the support screen, and leaves the filter through a pipe in the bottom of the filter vessel.
Deep-bed filters, like precoat filters, are cleaned by backwashing. Water is pumped through the distribution piping near the top of the filter. The flow rate of the water is kept high enough to lift the granulated charcoal or anthracite up into the free space. The water washes away the deposits that have accumulated. When the backwash cycle is completed, the flow is stopped, and the granules settle back down into the filter bed. The filter can then be put back into service.
Metal-Edged Filters
Metal-edged filters are used in the lubrication (oil) systems of many auxiliary units. A metaledged filter consists of a series of metal plates or disks. Turning a handle moves the plates or disks across each other in a manner that removes any particles that have collected on the metal surfaces. Some metal-edged type filters have magnets to aid in removing fine particles of magnetic materials.
Strainers
Strainers are fitted in many piping lines to prevent the passage of grit, scale, dirt, and other foreign matter, which could obstruct pump suction valves, throttle valves, or other machinery parts. One of the simplest and most common types of strainers found in piping systems is the Y-strainer, which is illustrated in Figure 23.
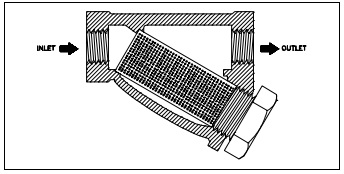
Figure 24 illustrates three additional common types of strainers. Part A shows a typical sump pump suction bucket strainer located in the sump pump suction line between the suction manifold and the pump. Any debris that enters the piping is collected in the strainer basket. The basket can be removed for cleaning by loosening the strongback screws, removing the cover, and lifting the basket out by its handle.
Part B of Figure 24 shows a duplex oil strainer commonly used in fuel oil and lubricating oil lines, where it is essential to maintain an uninterrupted flow of oil. The flow may be diverted from one basket to the other, while one is being cleaned.
Part C of Figure 24 shows a manifold steam strainer. This type of strainer is desirable where space is limited, because it eliminates the use of separate strainers and their fittings. The cover is located so that the strainer basket can be removed for cleaning.
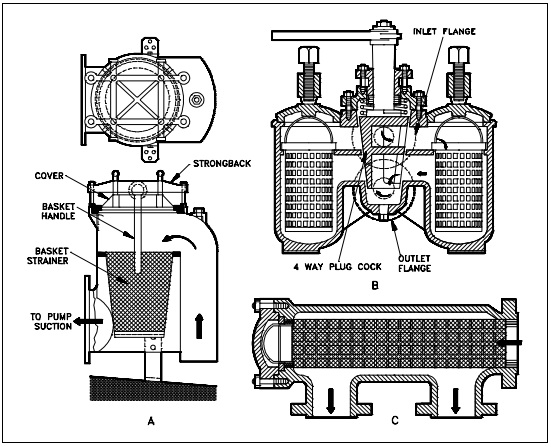
Backwashing
If the filter or strainer cannot be easily removed for cleaning, the system design will usually include a flowpath for backwashing. The backwashing of precoated filters has already been explained because it is more complex than a typical backwash. The intent of a backwash is to flow liquid in the opposite direction of normal flow, creating a pressure that pushes the debris off the strainer or filter. The debris is flushed to a waste tank or drain.
Normally, to establish a backwash lineup, the flowpath upstream of the inlet to the strainer or filter is closed, the flow path downstream of the outlet is closed, and a drain flowpath is opened.
The flush source is then opened and the flow goes into the outlet of the strainer or filter, through the strainer or filter, and exits the inlet to the backwash drain or waste tank, carrying the debris with it.