Steam System Basics
Why Steam?
There are three principal forms of energy used in industrial processes: electricity, direct-fired heat, and steam. Electricity is used in many different ways, including mechanical drive, heating, and electrochemical reactions. Direct-fired energy directly transfers the heat of fuel combustion to a process. Steam provides process heating, pressure control, mechanical drive, and component separation, and is a source of water for many process reactions.
Steam has many performance advantages that make it an indispensable means of delivering energy. These advantages include low toxicity, ease of transportability, high efficiency, high heat capacity, and low cost with respect to the other alternatives. Steam holds a significant amount of energy on a unit mass basis (between 1,000 and 1,250 British thermal units per pound [Btu/lb]) that can be extracted as mechanical work through a turbine or as heat for process use. Since most of the heat content of steam is stored as latent heat, large quantities of heat can be transferred efficiently at a constant temperature, which is a useful attribute in many process heating applications.
Steam is also used in many direct contact applications. For example, steam is used as a source of hydrogen in steam methane reforming, which is an important process for many chemical and petroleum refining applications. Steam is also used to control the pressures and temperatures of many chemical processes. Other significant applications of steam are to strip contaminants from a process fluid, to facilitate the fractionation of hydrocarbon components, and to dry all types of paper products.
The many advantages that are available from steam are reflected in the significant amount of energy that industry uses to generate it. For example, in 2006, U.S. manufacturers used about 4,762 trillion Btu of steam energy, which represents about 40% of the total energy used in industrial process applications for product output.
Steam used by energy intensive industries is especially significant. For example, in 2006 the forest products industry used approximately 2,117 trillion Btu of energy to generate steam, accounting for about 76% of the total onsite energy, excluding feedstocks used by this industry. The chemicals industry used approximately 1,504 trillion Btu of energy to generate steam, which represents about 47% of the total onsite energy, excluding feedstocks used in this industry. The petroleum refining industry used about 915 trillion Btu of energy to generate steam, which accounts for about 28% of this industry’s total onsite energy use, excluding feedstocks.2
Steam System Operation
This course uses four categories to discuss steam system components and ways to enhance steam system performance: generation, distribution, end use, and recovery. These four areas follow the path of steam as it leaves the boiler and returns via the condensate return system.
Generation
Steam is generated in a boiler or a heat recovery steam generator by transferring the heat of combustion gases to water. When water absorbs enough heat, it changes phase from liquid to steam. In some boilers, a superheater further increases the energy content of the steam. Under pressure, the steam then flows from the boiler or steam generator and into the distribution system.
Distribution
The distribution system carries steam from the boiler or generator to the points of end use. Many distribution systems have several take-off lines that operate at different pressures. These distribution lines are separated by various types of isolation valves, pressure-regulating valves, and, sometimes, backpressure turbines. A properly performing distribution system delivers sufficient quantities of high-quality steam at the right pressures and temperatures to the end uses. Effective distribution system performance requires proper steam pressure balance, good condensate drainage, complete and optimum insulation with regular repair and maintenance, and effective pressure regulation.
End Use
There are many different end uses of steam. Examples of steam’s diverse uses include process heating, mechanical drive, moderation of chemical reactions, and fractionation of hydrocarbon components. Common steam system end-use equipment includes heat exchangers, turbines, fractionating towers, strippers, and chemical reaction vessels.
In a heat exchanger, the steam transfers its latent heat to a process fluid. The steam is held in the heat exchanger by a steam trap until it condenses, at which point the trap passes the condensate into the condensate return system. In a turbine, the steam transforms its energy to mechanical work to drive rotating machinery such as pumps, compressors, or electric generators. In fractionating towers, steam facilitates the separation of various components of a process fluid. In stripping applications, the steam pulls contaminants out of a process fluid. Steam is also used as a source of water for certain chemical reactions. In steam methane reforming, steam is a source of hydrogen.
Recovery
The condensate return system sends the condensate back to the boiler. The condensate is returned to a collection tank. Sometimes the makeup water and chemicals are added here, while other times this is done in the deaerator. From the collection tank the condensate is pumped to the deaerator, which strips oxygen and non-condensable gases. The boiler feed pumps increase the feedwater pressure to above boiler pressure and inject it into the boiler to complete the cycle.
Figure 1 provides a general schematic description of the four principal areas of a steam system. The following sections discuss the components in these areas in greater detail. Consider opportunities to cascade heat recovery from exhaust gases to lower temperature process heating equipment. Develop procedures for regular operations, calibration, and maintenance of process sensors (i.e., pressure, temperature, and flow) and controllers.
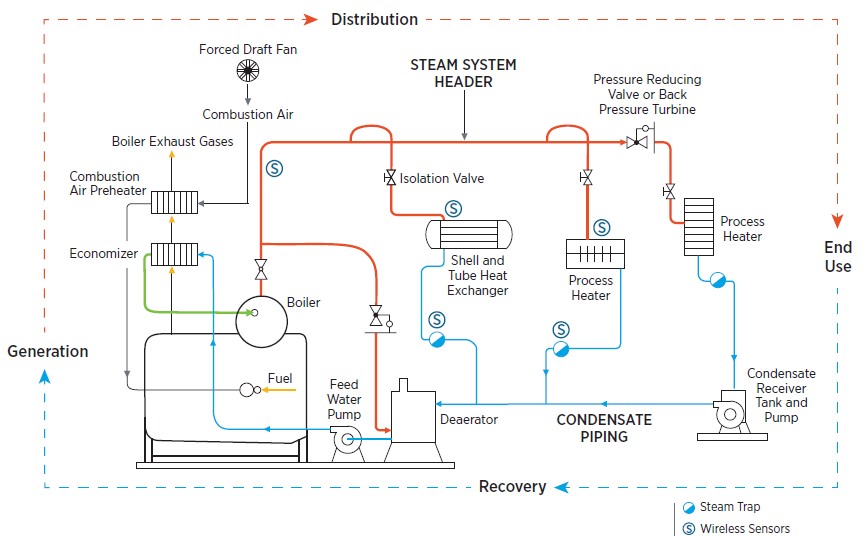
Steam System Basics – Recovery
The recovery components of a steam system collect and return condensate back to the generation part of the system. Condensate recovery provides thermal and water treatment benefits. Condensate that is not returned must be compensated for by the addition of makeup water, which is generally much cooler than condensate, however it is becoming less commonly available. Condensate temperature often exceeds 200°F, while makeup water temperature is typically between 50°F and 80°F. As a result, the enthalpy difference between condensate and makeup water is generally over 120 Btu/lb, an amount of energy that is often more than 10% of the energy in the boiler generated steam.
Additionally, makeup water is generally treated with chemicals that remove minerals and establish certain pH levels in the boiler water and in the system. Reducing the amount of makeup water added to the system reduces chemical use. Additionally, some of the treatment chemicals that are contained in condensate are problematic to a plant’s wastewater treatment facility. Industrial steam plants often extend across large areas. Recovering condensate from steam systems requires piping, collecting tanks, pumping equipment, and, in many cases, flash steam separators, meters, and filtration/cleanup equipment. However, the cost savings available from avoiding the purchase, treatment, and heating of makeup water often make investments in condensate recovery systems highly feasible.
Condensate Return Piping
Condensate return piping transports condensate as it drains from distribution and end-use equipment piping back to the boiler. Condensate piping should be adequately sized and insulated. Although the installation of larger pipe diameters is more expensive, larger pipes create less pressure drop for a given flow rate; this reduces the load on the condensate pumps. Larger pipe diameters also reduce the noise associated with condensate flow and are more suitable for carrying flash steam. Insulating the condensate piping helps to retain the thermal energy that provides much of the benefits of a condensate recovery system.
Insulation
Insulation provides energy savings and safety benefits. In terms of energy savings, insulation reduces heat loss from the condensate piping and recovery equipment surfaces, which can make the surrounding work environment more comfortable. Reducing this heat loss can also reduce the burden on the cooling systems that support surrounding workspaces. In terms of safety, insulation reduces the outer surface temperature of the piping, which lessens the risk of burns. Important insulation properties and characteristics of piping insulation are discussed in the Distribution section. See Tip Sheet #2, Insulate Steam Distribution and Condensate Return Lines at: manufacturing.energy.gov.
Condensate Receiver Tanks
Condensate receiver tanks collect and store condensate. These tanks are usually located remotely around the condensate system and are configured in conjunction with condensate pumps, as shown in Figure 14. Condensate flows can be highly variable due to changes in steam demand, especially during system start-ups. Receiver tanks minimize the effects of this flow variability on condensate pumps by providing storage, which maintains a minimum water level that prevents downstream condensate pumps from running dry. Since many condensate pumps are centrifugal types, it is important to keep a certain suction pressure to prevent cavitation damage. By maintaining a minimum condensate level, receiver tanks provide enough static pressure to avoid cavitation.
Most systems also contain a large condensate receiver tank that collects all the condensate returned from the system. This tank may also be used to store pretreated water.
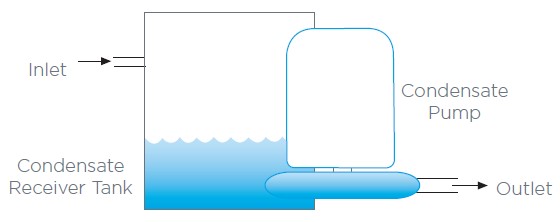
Condensate Pumps
Condensate pumps move condensate from receiver tanks back to the boiler room. Condensate pumps can be driven by electric motors, steam, or compressed air, depending on the availability of these sources. Motor-driven condensate pumps are usually centrifugal type pumps. In many cases, receiver tanks and motor-driven pumps are packaged together and equipped with a control system that de-energizes the pump under low water level conditions. Steam or compressed air powered condensate pumps are used where electrical pumps would not be suitable, and are generally pressure-powered pumps.
Condensate pumps also can be important to the performance of end-use equipment. Effective use of condensate pumps can eliminate condensate backup into end-use equipment, improving process control and reducing potential equipment problems from condensate acidification and water hammer.
Flash Steam Vessels
Flash steam vessels allow the recovery of steam from condensate lines, as illustrated in Figure 15. By removing steam from the condensate system, flash steam vessels provide an efficient source of steam to low-pressure end uses. For example, 250°F condensate has a saturation pressure of about 15 psig. Consequently, steam that is separated by flash steam vessels can be used in low-pressure steam applications such as space heating and preheating.
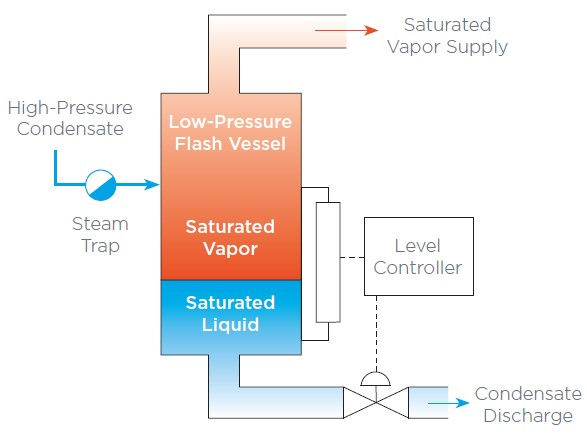
Condensate Meters
Condensate meters measure the flow rate of condensate in the return system. Knowing the condensate flow rate can be helpful in monitoring the condensate system and the condition of steam traps. Condensate meters are often inline rotary types, relying on turbine or scroll rotation to measure flow rate.
Filtration/Cleanup Equipment
In many systems, the flow of steam and condensate picks up rust, scale, and trace contaminants that are either carried over from the boiler or that form in carbon steel piping and on copper alloy heat exchange surfaces. Although strainers and filters are used to catch the particulate matter, some contaminants are dissolved in the condensate and can cause problems if returned to the boiler. In systems that require a high level of cleanliness, condensate polishers are used. Condensate polishers use ion exchange to remove these contaminants, preventing the redeposition of these contaminants on boiler surfaces.
Steam System Basics – End Use
Steam system end-use equipment transfers steam energy into other forms of useful energy. Common end-use equipment includes heat exchange devices to transfer thermal energy and turbines to recover mechanical energy. In manufacturing industries, steam end uses often directly support production, making their performance and reliability essential to plant productivity. Improvements in end-use efficiency and effectiveness also tend to result in better performance and increased reliability. There is a wide range of end-use equipment, largely because of the advantages of steam that are discussed in the Introduction. Some of the major end-use components are discussed in this section.
For the purposes of this discussion, steam end-use equipment is grouped into three basic categories:
- Industries of key end-use equipment in Energy Intensive Industries
- Conditioning and control equipment
- Additional equipment
The key equipment category includes the largest uses of steam in those industries. Although facilities use steam for other services as well, the key end uses account for the largest amount of steam use. The conditioning equipment category includes equipment that facilitates the effective use of steam. The additional equipment category includes equipment that is used in other industries and, though significant, does not account for most of the steam use in energy intensive industries.
Key End-Use Equipment in Energy Intensive Industries
In the three industries of forest products, petroleum refining, and chemicals, steam accounts for the largest amount of end-use energy. In integrated steel production, steam represents a significant amount of end-use energy and is used to generate most of that industry’s on-site electric power. Table 1 provides a list of key steam-supplied end-use equipment for energy intensive industries.
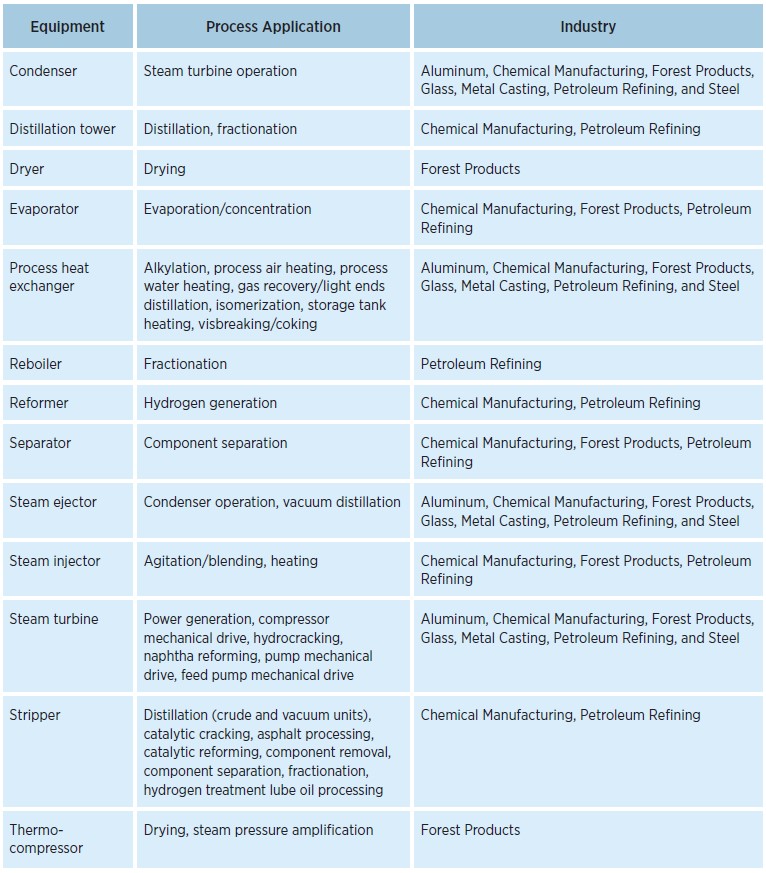
Condensers
In steam applications, condensers are associated with condensing steam turbines and with multiple stage ejector systems. In steam turbine applications, condensers typically operate under a vacuum. They remove energy from the exhaust steam, allowing it to be recovered as condensate. In steam ejector applications, condensers increase the effectiveness of the ejectors by condensing both the motive steam and condensables pulled from the process, reducing the amount of motive steam required.
Condensers can be surface type or barometric. Surface condensers are supplied with cooling water that circulates through condenser tubes, providing a cool surface area that causes steam condensation. The condensate is typically collected in a condensate well, and pumped into the condensate return system. Barometric condensers rely on direct contact between the cooling water and the steam. In petroleum refining and chemical manufacturing applications, condensers are also used to condense components from gaseous mixtures. In these applications, the condensers use a cooling medium to extract energy from the gases and collect the condensed components.
Distillation Towers
The petroleum refining and chemical manufacturing industries use large amounts of steam to facilitate the separation of crude oil or chemical feedstocks into various components. This separation process relies on differences in the boiling points of these hydrocarbon components. Fractionating towers use a furnace to heat crude oil above 700°F. As the volatile components boil off and rise up the tower, they cool and condense on trays. Steam is injected into the bottom of these towers to reduce the partial pressures of the hydrocarbons, which facilitates their separation, and to reduce coke formation on tray and tower surfaces.
Dryers
Dryers reduce the water content of a solid. Dryers account for the largest end use of steam in the pulp and paper industry.9 The chemical manufacturing, textiles, and food processing industries also use large amounts of steam for drying. Dryers can be indirect or direct. Indirect dryers remove moisture thermally as energy is transferred from condensing steam, flue gases, or high temperature process fluid to the product being dried. Common indirect dryer types are coil and rotating drum. Direct dryers use hot gases that have been heated with steam or flue gases to directly contact and dry a product.
Dryers, like evaporators, can be arranged in multiple- stage configurations. Multiple-stage steam dryers use
a cascading set of steam pressures, allowing steam released from an upstream stage to supply steam to
the next stage. In many multiple-stage dryers, thermo-compressors are used to increase the steam pressure of downstream-effect stages.
Evaporators
Evaporators reduce the water content of a liquid, generally by heating it with steam to concentrate the product. Evaporators are used extensively in industries such as food processing, chemical manufacturing, steel, forest products, and textiles.
In most cases, evaporators are shell and tube heat exchangers with the steam on the shell side and the product being concentrated in the tubes. Evaporators can be single effect or multiple effect. A single-effect evaporator uses steam at one set of pressure and temperature conditions to boil off the vapor from a product. Multiple-effect evaporators take the vapor produced from one evaporator and use it to heat the product in a lower-pressure evaporator. Multiple-effect evaporators are generally more efficient at concentrating a fluid than single-effect evaporators.
Heat Exchangers
Heat exchangers transfer thermal energy from one fluid to another. In manufacturing facilities, steam is a common source of heat for many reasons, some of which are discussed in the Introduction. There is a wide range of heat exchanger designs that use steam, largely due to the wide range of products that are heated with steam.
Many process and product considerations must be incorporated into the selection of a heat exchanger. Some basic heat exchanger types are discussed below, including:
- Tubular
- Plate and frame
- Jacketed
- Coil.
Tubular heat exchanger. Tubular heat exchangers are tube bundles that are surrounded by the heated or heating medium. This type of heat exchanger includes finned tube and shell and tube designs as shown in Figure 10. Finned tube heat exchangers are often used to heat air for drying and space heating applications. Shell and tube heat exchangers are often used for liquid heating and evaporation. Since the tube side of shell and tube heat exchangers can be designed to withstand high pressures, sometimes exceeding 1,500 psig, heat exchangers of this type are often used in high-temperature and high-pressure applications.

Plate and frame heat exchanger. In plate and frame heat exchangers, the two heat exchange fluids are separated by plates. The plates are corrugated, or ridged, as shown in Figure 11, to increase the surface area available for heat transfer. Plate and frame heat exchangers are often used in low-viscosity applications, where the risk of clog-ging is less severe. The plate ends are typically sealed by covers with gaskets covers that can be removed to allow disassembly and cleaning. This heat exchanger type is used when temperatures and pressures are moderately low, typically below 300°F and 370 psi. Plate and frame heat exchangers also have a common design variation that has the plates welded or brazed together. This allows higher temperature service but eliminates the possibility of mechanical cleaning.
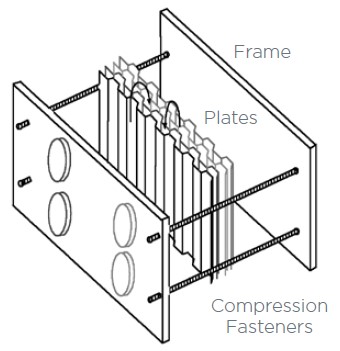
Jacketed heat exchangers. Jacketed heat exchangers use an enclosure to surround the vessel that contains the heated product. A common example of a jacketed heat exchanger is the jacketed kettle. A representation of a jacketed heat exchanger is shown in Figure 12. Jacketed heat exchangers are practical for batch processes and for product types that tend to foul or clog tube bundles or coils.

Heat Exchanger
Coil heat exchangers. Coil heat exchangers characteristically use a set of coils immersed in the medium that is being heated. Coil heat exchangers are generally compact, offering a large heat transfer area for the size of the heat exchanger.
Reboilers
Reboilers are typically used in distilling processes to increase component separation. Reboilers use heat, often provided by steam, to evaporate the volatile components of a product that has been drawn from a fractionating tower. These volatile components are sent downstream for further processing. The residual components are sent back into the fractionating tower or sent on to a vacuum distillation process. There are several types of reboilers, including jacketed kettle, kettle, internal reboiler, and thermosyphon reboiler. These designs differ from one another in the way the product is heated with steam.
Reformers
Steam reformers are used to generate hydrogen, typically from a hydrocarbon feedstock such as methane (the largest component of natural gas). In turn, hydrogen is used in many petroleum refining and chemical manufacturing processes. Reformers use steam for both energy and as a source of hydrogen. Steam is injected with the hydrocar-bon feedstock to initiate the following reaction:
Reformers often have secondary stages that are used to convert the carbon monoxide to carbon dioxide and additional hydrogen. Although large amounts of steam are used throughout the reforming processes, steam is also generated by the reformers and is sometimes exported for other uses.
Steam Ejectors
Steam ejectors use steam flow through a nozzle to create a vacuum (similar in operation to thermocompressors). They are used in several different types of system applications and process equipment. Low-pressure conditions promote the evaporation of liquids at reduced temperatures.
Consequently, many chemical manufacturing processes use steam ejectors to increase the concentration of a product. In petroleum refining, steam ejectors are commonly used in the vacuum distillation of heavy hydrocarbon products. Steam ejectors are also used to initiate and maintain vacuum condition in the condensers of condensing turbines.
Steam Injectors
Steam injectors are used to inject steam directly into a tank or a pipe containing a process fluid, generally for heating purposes. Many injector types use a nozzle and a diffuser to pull process fluid into the steam before the mixture is injected into the process fluid, to promote an even distribution of heat. Important performance characteristics of injectors include accurate control of the amount of steam injected and effective mixing of the steam and process.
Steam Turbines
Steam turbines are used to drive electric generators or other rotating machinery such as compressors, pumps, and fans. Steam turbines are used in many different system designs, depending on the relative requirements for steam, electricity, or other mechanical loads. Steam turbines pro-vide an effective means of stepping down steam pressure while extracting mechanical work.
Some turbines have interstage take-offs that allow steam to be extracted at various pressures before reaching the turbine exhaust. These extractions provide flexibility in meeting competing requirements of both the steam system and the mechanical load. For example, if the turbine is connected to an electric generator, adjusting the amount of extracted steam can allow more or less electric power to be generated, while making respectively less or more steam available to the plant.
Backpressure turbines. Backpressure turbines exhaust steam at pressures that are higher than atmospheric, and the exhaust steam is then used for other services. By extracting mechanical work from steam, backpressure tur-bines can provide an efficient means of supplying lower-pressure steam from a high-pressure header.
Condensing turbines. Condensing turbines exhaust steam to vacuum (sub-atmospheric) conditions. This steam is condensed in a heat exchanger, referred to as a condenser, and transferred to the condensate return system. Condensing turbines typically require a source of cooling water to condense the steam.
Strippers
Steam strippers are used to remove contaminants from a solution. Strippers are commonly found in petroleum refining and chemical manufacturing applications, where process solutions contain components that have different boiling points and removal of one or more of the components is necessary. Injecting steam into the process solution lowers the partial pressure of volatile components, allowing some of them to vaporize and get transported away with the steam. Steam can also raise the temperature of the mixture, lowering the solubility of the objectionable material and causing it to strip off with the steam. Often, the steam and the contaminants are condensed and separated, allowing recovery of the condensate and disposal or further processing of the contaminant.
Thermocompressors
Thermocompressors combine high-pressure and low-pressure steam to form an intermediate-pressure steam supply (see Figure 13). Often the low-pressure steam does not have enough energy to be feasibly used; however, discharging it to the condensate return system can be an unnecessary energy loss. Thermocompressors use a high-pressure steam source to recover the energy from this low-pressure source, providing an intermediate steam supply that can be feasibly used.
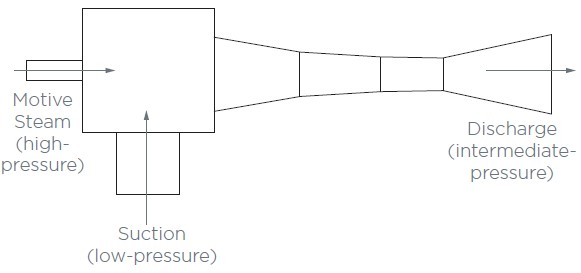
Conditioning and Control Equipment
Conditioning equipment is generally used to improve the performance of, or to protect the end-use equipment. For example, desuperheaters are often used to control the energy of a steam supply to end-use equipment to reduce the risk of damage to the equipment or to effectively improve temperature control of the process.
Desuperheaters. The purpose of a desuperheater is to remove the superheat from steam. The majority of heating and process equipment performs more efficiently using saturated rather than super-heated steam. Desuperheaters inject a very fine mist of high-purity water, such as condensate, into the steam flow. The superheated vapor gives up heat to the water mist, and by doing so, reduces its temperature.
Vacuum breakers. Vacuum conditions can develop in a steam system when steam flow into a component or a branch is throttled or shut off. If the rate of downstream steam use exceeds the steam supply, the pressure decreases and vacuum conditions can form. Vacuum conditions also result when the load on the heat exchanger is significantly less than the heat exchanger capacity. If the pressure in the heat exchanger drops too far, the condensate will not drain from the trap due to a higher pressure on the trap’s downstream side. If uncorrected, the condensate level will rise in the heat exchanger, reducing the available heat transfer area and increasing the risk of corrosion by condensate. Vacuum breakers are pressure-controlled devices that essentially vent a heat exchanger or system branch in which a vacuum has formed. By allowing in air when they open, vacuum breakers restore pressure and allow the condensate to drain.
Air vents. Before start-up, the steam system contains air that must be removed. The presence of air in a steam system reduces heat transfer effectiveness and promotes condensate corrosion. Air vents remove this air. Air vents are often thermostatic devices, similar to thermostatic steam traps that rely on the temperature difference between air and steam. When exposed to the lower temperature air in the system side, the vent opens. As the higher temperature steam reaches the vent, it closes, preventing the escape of steam.
Traps. Steam traps are important to the performance of end-use equipment. Traps provide for condensate removal with little or no steam loss. If the traps do not function properly, excess steam will flow through the end-use device or the condensate will back up into it.
Excess steam loss will lead to costly operation, while condensate backup will promote poor performance and may lead to water hammer. Traps can also remove noncondensable gases that reduce heat exchanger effectiveness. There are several different types of steam traps, which are dis-cussed in the Distribution section of this course.
Insulation
End-use equipment, such as heat exchangers and turbines, should generally be insulated due to the significant heat loss that the surface areas of this equipment can pro-vide. The various types of insulation are discussed in the Distribution section. Where end-use equipment requires frequent inspection or maintenance, removable insulation should be considered.
Additional Equipment
The additional equipment category refers to end uses of steam throughout industry; although still significant, these generally account for less steam energy than key end uses.
Absorption chillers. Absorption chillers provide cooling using an interesting variation of the vapor compression cycle. Instead of a compressor, which is generally used in chillers, absorption chillers exploit the ability of one sub-stance to absorb a refrigerant at one temperature and then release it at another. In ammonia-based systems, water is the absorbent and ammonia is the refrigerant. In lithium bromide-based systems, lithium bromide is the absorbent, while water is the refrigerant. An absorption chiller uses a pump instead of a compressor to increase refrigerant pressure. Once it is at the higher pressure, the absorbent/refrigerant solution is heated, often with steam, which releases the refrigerant. Although absorption chillers generally have lower coefficients of performance (COP) (indicating lower thermodynamic efficiency) than traditional chillers, they use less electric power per ton of cooling and are well suited for use with steam systems.
Humidifiers. Humidifiers inject steam into an air or other gas source to increase its water vapor content. In humidification, steam is used as a source of both water and energy. Humidification applications are found in the chemical manufacturing industry where control of ambient temperature and moisture content are critical for product quality.
Preheat/Reheat air handling coils. While steam is occasionally used in space heating applications to preheat and reheat air, there are other more efficient methods of con-trolling space humidity levels. In many HVAC systems, the conditioned air must have both its temperature and humidity adjusted. In preheat applications, steam is used to heat an air supply, which is typically a mixture of return air and outside air. The air is then conditioned to achieve a certain humidity and temperature. In reheat applications, the air is cooled to a particular dew point to remove water and achieve a desired humidity. As a result, before the air is delivered back to the workspaces, steam coils must reheat the process air stream up to the proper temperature. In both reheat and preheat applications, finned tube heat exchangers are generally used.
Tracing. In tracing applications, steam is used to maintain the temperature of a fluid in a pipe. A common application of tracing lines is to prevent the freezing of a process fluid in piping that runs outside of a temperature-controlled area. Since tracing lines are exposed to freezing conditions, proper insulation, steam flow, and condensate drain-age are essential to prevent freezing of the tracing lines as well as the process piping.
Meters. Steam meters are used to measure steam flow, and are important for tracking the steam use of a particular part of a steam system or a particular end use. Discussion of different meter types is provided in the Steam Generation section.
Steam System Basics – Distribution
The distribution system transports steam from the boiler to the various end uses. Although distribution systems may appear to be passive, in reality, these systems regulate the delivery of steam and respond to changing temperature and pressure requirements. Consequently, proper performance of the distribution system requires careful design practices and effective maintenance. The piping should be properly sized, supported, insulated, and configured with adequate flexibility. Pressure-regulating devices such as pressure-reducing valves and backpressure turbines should be configured to provide proper steam balance among the different steam headers. Additionally, the distribution system should be configured to allow adequate condensate drainage, which requires adequate drip leg capacity and proper steam trap selection. Steam distribution systems can be broken down into three different categories: buried pipe, above-ground, and building sections, and selection of distribution components (piping, insulation, etc.) can vary depending on the category.
Selected Energy Efficiency Practices for Steam Distribution Systems
- Validate energy intensity baseline and improvements with ASME Steam System Assessment Standard.
- Locate improved profit opportunities with data sources, such as wireless sensors, near your largest steam traps.
- Configure distribution systems to include adequate condensate drainage in startup and operating modes, for flash steam recovery and for optimized condensate return to the boiler.
Piping
Steam piping transports steam from the boiler to the end-use services. Important characteristics of well-designed steam system piping are that it is adequately sized, configured, and supported. Installation of larger pipe diameters may be more expensive, but can create less pressure drop for a given flow rate. Additionally, larger pipe diameters help to reduce the noise associated with steam flow. As such, consideration should be given to the type of environment in which the steam piping will be located when selecting the pipe diameter. Important configuration issues are flexibility and drainage. With respect to flexibility, piping (especially at equipment connections), needs to accommodate thermal reactions during system start-ups and shutdowns. Additionally, piping should be equipped with a sufficient number of appropriately sized drip legs to promote effective condensate drainage. Additionally, the piping should be pitched properly to promote the drainage of condensate to these drip lines. Typically, these drainage points experience two very different operating conditions, normal operation and start-up; both load conditions should be considered in the initial design.
Insulation
Thermal insulation provides important safety, energy savings, and performance benefits. In terms of safety, insula-tion reduces the outer surface temperature of the steam piping, which lessens the risk of burns. A well-insulated system also reduces heat loss to ambient workspaces, which can make the work environment more comfortable. Consequently, the energy saving benefits include reduced energy losses from the steam system and reduced burden on the cooling systems that remove heat from workspaces. In addition to its safety and energy benefits, insulation increases the amount of steam energy available for end uses by decreasing the amount of heat lost from the distribution system. See the Mechanical Insulation Design Guide, at www.wbdg.org/midg, for more on steam system insulation.
Important insulation properties include thermal conductivity, strength, abrasion resistance, workability, and resistance to water absorption. Thermal conductivity is the measure of heat transfer per unit thickness. Thermal conductivity of insulation varies with temperature; consequently, it is important to know the right temperature range when selecting insulation. Strength is the measure of the insulation’s ability to maintain its integrity under mechanical loads. Abrasion resistance is the ability to withstand shearing forces. Workability is a measure of the ease with which the insulation is installed. Water absorption refers to the tendency of the insulation to hold moisture. Insulation blankets (fiberglass and fabric) are commonly used on steam distribution components (valves, expansion joints, turbines, etc.) to enable easy removal and replacement for maintenance tasks.
Some common insulating materials used in steam systems include calcium silicate, mineral fiber, fiberglass, perlite, and cellular glass. The American Society for Testing and Materials (ASTM) provides standards for the required properties of these and other insulation materials. Additionally, the North American Insulation Manufacturers Association (NAIMA) has developed a software program titled 3E Plus that allows users to determine the energy losses associated with various types and thicknesses of insulation. The 3E Plus program facilitates the assess-ment of various insulation systems to determine the most cost-effective solution for a given installation. See Section 2, for more about 3E Plus Insulation software, which can help steam users assess insulation opportunities.
Selected Energy Efficiency Practice for Valves
- Locate improved profit opportunities with data sources, such as wireless sensors, near your largest valves.
- Use backpressure turbines, where possible, to avoid energy losses associated with pressure reductions through valves.
Valves
In steam systems, the principal functions of valves are to isolate equipment or system branches, to regulate steam flow, and to prevent over-pressurization. The princi-pal types of valves used in steam systems include gate, globe, swing check, pressure reducing, and pressure relief valves. Gate, globe, and swing check valves typically isolate steam from a system branch or a component.
Pressure reducing valves (PRV) typically maintain certain downstream steam pressure conditions by controlling the amount of steam that is passed. These reducing valves are often controlled by transmitters that monitor downstream conditions. Pressure relief valves release steam to prevent over-pressurization of a system header or equipment.
Steam Separators
In some steam systems, wet steam is generated. This wet steam contains water droplets that can reduce the effectiveness of the steam system. Water droplets erode piping elbows, turbine blades and passages, and pressure reducing valves, thus reducing efficiency and life. Furthermore, liquid water can significantly reduce heat transfer rates in heat exchange components, as well as result in water ham-mer. Removing water droplets before they reach end-use equipment is necessary.
Steam separators remove water droplets, generally relying on controlled centrifugal flow. This action forces the entrained moisture to the outer wall where it is removed from the separator. The means of moisture removal could be a steam trap or a drain. Some manufacturers include the trap as an integral part of the unit. Additional accessories include water gauge connections, thermometer connections, and vent connections.
Steam separators can be installed in either a horizontal or vertical line. They are capable of removing 99% of particulate entrainment 10 microns and larger over a wide range of flows. Separators are often designed in accordance with ASME Code, Section VIII, Division 1, with pressures to 300 psig.
Steam Accumulators
A steam accumulator is a large insulated pressure vessel, partially filled with hot water (saturated liquid). When steam supply exceeds demand, the excess high-pressure steam is charged into the accumulator through special charging nozzles. The steam is condensed, giving up its latent heat, to raise the pressure, temperature, and heat con-tent of the water body. When the steam demand exceeds the supply, the pressure in the accumulator drops and the additional required steam flashes from the water, taking back the heat previously stored. A simple system of control valves and check valves regulates the charging and discharging. The excess steam is charged quietly and smoothly, and when steam is needed, it is available with the speed of a control valve operation. There is also an accumulator design that stores hot water for use as boiler feedwater.
Steam Traps
Steam traps are essential for proper distribution system performance. During system start-ups, traps allow air and large quantities of condensate to escape. During system operation, the traps allow collected condensate to pass into the condensate return system, while minimizing the accompanying loss of steam. There are three primary types of traps: thermostatic, mechanical, and thermodynamic.
Selected Energy Efficiency Practices for Steam Traps
- Validate energy intensity improvement opportunities with ASME Steam System Assessment Standard.
- Improve profits with data from wireless sensors at steam traps by continuously monitoring and alarming for leakage. If you try one, chances are you’ll want more. Favorable returns on investments have been reported in their use.
- Several types of steam traps, including mechanical traps, are highly sensitive to correct installation arrangement and even insulation quantity to operate properly. Follow the installation and insulation guidance of the trap manufacturer carefully.
Thermostatic Traps
Thermostatic traps use temperature differential to distinguish between condensate and live steam. This differential is used to open or close a valve. Under normal operating conditions, the condensate must cool below the steam temperature before the valve will open. Common types of thermostatic traps include bellows and bimetallic traps.
Bellows traps. Bellows traps include a valve element that expands and contracts in response to temperature changes. Often a volatile chemical such as alcohol or water is inside the element. Evaporation provides the necessary force to change the position of the valve. At start-up, the bellows trap is open due to the relative cold condition. This operating condition allows air to escape and provides maximum condensate removal when the load is the highest. Bellows traps can fail either open or closed. The configuration of a bellows steam trap is shown in Figure 5.
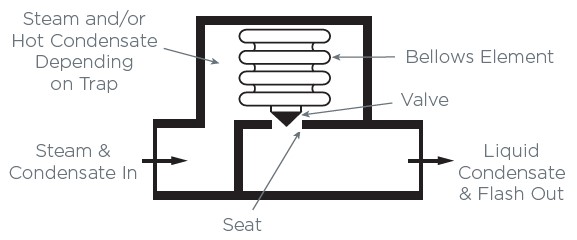
Bimetallic traps. Bimetallic traps rely on the bending of a composite strip of two dissimilar metals to open and close a valve. Air and condensate pass freely through the valve until the temperature of the bimetallic strip approaches the steam temperature. After steam or relatively hot condensate heats the bimetallic strip and causes it to close the valve, the trap remains shut until the temperature of the condensate cools sufficiently to allow the bimetallic strip to return to its original shape and thereby open the valve. Bimetallic traps can fail in either the open or closed position. The con-figuration of a bimetallic steam trap is shown in Figure 6.

Mechanical Traps
Mechanical traps use the difference in density between condensate and live steam to produce a change in the position of a float or bucket. This movement causes a valve to open or close. There are a number of mechanical trap designs that are based on this principle. They include ball float, float and lever, inverted bucket, open bucket, and float and thermostatic traps.
Ball float traps. Ball float traps rely on the movement of a spherical ball to open and close the outlet opening in the trap body. When no condensate is present, the ball covers the outlet opening, thereby keeping air and steam from escaping. As condensate accumulates inside the trap, the ball floats and uncovers the outlet opening. This movement allows the condensate to flow continuously from the trap. Unless they are equipped with a separate air vent, ball float traps cannot vent air on start-up.
Float and lever traps. Float and lever traps are similar in operation to ball float traps except the ball is connected to a lever. When the ball floats upward due to accumulation of condensate inside the trap body, the attached lever moves and causes a valve to open. This action allows condensate to continuously flow from the trap. If the condensate load decreases and steam reaches the trap, downward ball movement causes the valve to close, thereby keeping steam from escaping. Unless they are equipped with a separate air vent, float and lever traps cannot vent air on start-up. See the discussion on float and thermostatic traps.
Inverted bucket traps. Inverted bucket traps are some-what more complicated than float and lever traps. At start-up, the inverted bucket inside the trap is resting on the bottom of the trap body and the valve to which the bucket is linked is wide open. The trap is initially filled with condensate. As steam enters the trap and is captured inside the bucket, it causes the bucket to move upward. This upward movement closes the valve and keeps steam from escaping. When the condensate collects and cools the steam, the bucket moves downward. This movement causes the valve to open, thereby allowing the condensate to escape. Unlike closed float traps, inverted bucket traps have intermittent discharge. These traps can be depleted of their condensate seal when applied in superheated steam service. If this occurs, the trap will continuously discharge live steam. This trap type is not recommended for superheated steam service, unless special installation conditions are met. The configuration of an inverted bucket steam trap is shown in Figure 7.
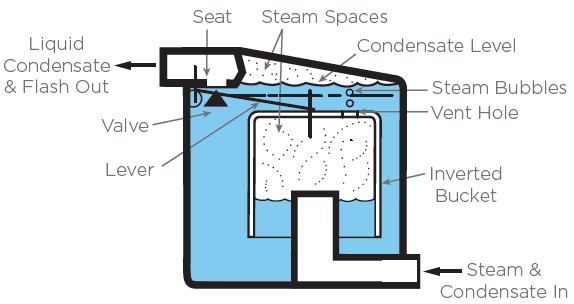
Open bucket traps. Open bucket traps consist of an upright bucket that is attached to a valve. At start-up, the bucket rests on the bottom of the trap body. In this position, the valve is wide open. As condensate accumulates in the trap body on the outside of the bucket, the bucket floats upward, causing the valve to close. When sufficient condensate accumulates outside the bucket, it spills over the top and fills the inside of the bucket. At this time, the bucket sinks, causing the valve to open. This trap is also prone to failure when applied in superheated steam service because of the loss of the condensate seal. Like inverted bucket traps, open bucket traps have intermittent discharge.
Float and Thermostatic (F&T) traps. Float and thermostatic (F&T) traps are similar to float and lever traps except they include a thermostatic element that allows air to be discharged at start-up and during operation. The thermostatic elements used in these traps are the same as those used in thermostatic traps. The configuration of a float and thermostatic steam trap is shown in Figure 8.

Thermodynamic Traps
Thermodynamic traps use the difference in kinetic energy (velocity) between condensate and live steam to operate a valve. The disc trap is the most common type of thermodynamic trap, but piston or impulse traps are sometimes used.
Disc traps. Disc traps use the position of a flat disc to control steam and condensate flow. When condensate flows through the trap, the disc is raised, thereby causing the trap to open. As steam and air pass through the trap, the disc moves downward. The force that causes the disc to move downward is generated by the difference in pressure between the low-velocity steam above the disc and the high-velocity steam that flows through the narrow gap beneath the disc. Disc traps commonly have an intermit-tent discharge and, when they fail, they normally fail open. The configuration of a disc steam trap is shown in Figure 9. Generally, the air removal capability of this trap type is poor unless equipped with additional components (like the float and thermostatic trap).

Piston traps. Piston or impulse traps utilize the heat energy in hot condensate, and the kinetic energy in steam, to open and close a valve. Like disc traps, piston traps are phase detectors that sense the difference between a liquid and gas or vapor. They continuously discharge any air and condensate. Their primary failure mode is open.
Lever traps. Lever traps are a variation of the thermodynamic piston trap. They operate on the same principal as a piston trap but with a lever action to pass large amounts of condensate and air on a continuous basis. Their primary failure mode is open.
Orifice traps. Orifice traps are of two basic types: orifice plate and short tube. Both trap types operate under the exact same principles. A simple orifice plate steam trap consists of a thin metal plate with a small-diameter hole (orifice) drilled through the plate. When installed, condensate that accumulates is continuously removed as the steam pressure forces the condensate through the orifice. During conditions when no condensate is present, a limited amount of steam flows through the orifice. The report Review of Orifice Plate Steam Traps in the Where to Find Help section provides information for making informed decisions about when orifice plate steam traps should be considered for use in new or existing steam systems.
Steam Meters
The use of flowmeters within the distribution system can provide important data for monitoring the efficiency of
a process or an end use. Tracking the amount of steam required can be particularly useful in benchmarking efforts. The types of steam flowmeters are discussed in the Generation Section. The DOE Federal Energy Management Program has a Metering Best Practices Guide, Release 2.0, which includes steam use.
Steam System Basics – Generation
The generation part of a steam system uses a boiler to add energy to a feedwater supply to generate steam.
The energy is released from the combustion of fossil fuels or from process waste heat. The boiler provides a heat transfer surface (generally a set of tubes) between the combustion products and the water. The most important parts of the generating system include the boiler, the fuel supply, combustion air system, feedwater system, and exhaust gases venting system. These systems are related because problems or changes in one generally affect the performance of the others. DOE research partnerships with the industry have resulted in high-efficiency burners and other technologies for industrial boilers.
Boilers
There are two basic types of boilers: firetube and water-tube. The fundamental difference between these boiler types is which side of the boiler tubes contain the combustion gases or the boiler water/steam.
Firetube boilers. In firetube boilers, the combustion gases pass inside boiler tubes, and heat is transferred to water between the tubes and the outer shell. A representative firetube boiler is shown in Figure 2. Scotch marine boilers are the most common type of industrial firetube boiler. The Scotch marine boiler is an industry workhorse due to low initial cost and advantages in efficiency and durability.

Scotch marine boilers are typically cylindrical shells with horizontal tubes configured such that the exhaust gases pass through these tubes, transferring energy to boiler water on the shell side.
Selected Energy Efficiency Practices for Steam Systems and Boiler Operations
- Validate energy intensity improvements with Superior Energy Performance and ANSI or ISO Energy Management Standard.
- Locate savings opportunities and validate energy savings with data from interval monitoring or metering.
- Continuously maintain efficient systems by matching boiler capacities and operations to process load and loading cycles.
Scotch marine boilers contain relatively large amounts of water, which enables them to respond to load changes with relatively little change in pressure. However, since the boiler typically holds a large water mass, it requires more time to initiate steaming and more time to accommodate changes in steam pressure. Also, Scotch marine boilers generate steam on the shell side, which has a large surface area, limiting the amount of pressure they can generate. In general, Scotch marine boilers are not used where pressures above 300 pounds per square inch gauge (psig) are required. Today, the biggest firetube boilers are over 1,500 boiler horsepower (about 50,000 pounds per hour4).
Firetube boilers are often characterized by their number of passes, referring to the number of times the combustion (or flue) gases flow the length of the pressure vessel as they transfer heat to the water. Each pass sends the flue gases through the tubes in the opposite direction. To make another pass, the gases turn 180 degrees and pass back through the shell. The turnaround zones can be either dryback or waterback. In dryback designs, the turnaround area is refractory-lined. In waterback designs, this turn-around zone is water-cooled, eliminating the need for the refractory lining.
Watertube Boilers. In watertube boilers, boiler water passes through the tubes while the exhaust gases remain in the shell side, passing over the tube surfaces. A representative watertube boiler is shown in Figure 3. Because tubes can typically withstand higher internal pressure than the large chamber shell in a firetube, watertube boilers are used where high steam pressures (3,000 psi, sometimes higher) are required. Watertube boilers are also capable of high efficiencies and can generate saturated or superheated steam. In fact, the ability of watertube boilers to generate superheated steam makes these boilers particularly attractive in applications that require dry, high-pressure, high-energy steam, including steam turbine power generation. Another type of watertube boiler is a coil type watertube boiler shown in Figure 4.

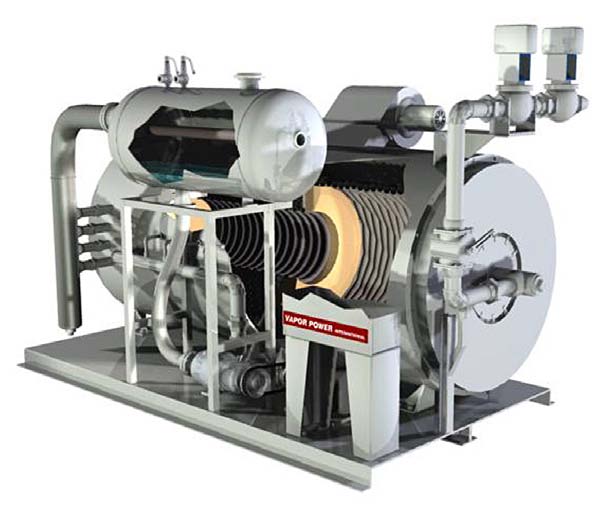
The performance characteristics of watertube boilers make them highly favorable in process industries, including chemical manufacturing, pulp and paper manufacturing, and refining. Although firetube boilers account for the majority of boiler sales in terms of units, watertube boilers account for the majority of boiler capacity.
Waste Heat Recovery Boiler (WHRB). These boilers may be either firetube or watertube design and use heat that would otherwise be discarded to generate steam. Typical sources of heat for WHRBs include exhaust gases or high-temperature products from an external manufacturing process in refineries and chemical manufacturing facilities, or combustion of a waste fuel in the boiler furnace.
Heat Recovery Steam Generators (HRSGs). HRSGs transfer energy from the exhaust of a gas turbine to an unfired or supplementary fired heat-recovery steam generator to produce steam. Exhaust gases leave the gas turbine at temperatures of 1,000°F (538°C) or higher and can represent more than 75% of the total fuel energy input. This energy can be recovered by passing the gases through a heat exchanger (steam generator) to produce hot water or steam for process needs. If the amount of steam needed by the process exceeds the amount produced by simple heat recovery, then supplementary fuel can be burned in an inline duct burner between the gas turbine and the HRSG.
Superheaters. Superheaters add energy to steam, resulting in a steam temperature that exceeds the saturation temperature at a specific pressure, which is typically known as superheated steam. Superheaters can be convective or radiant. Radiative superheaters rely on the energy transferred directly from the combustion flame to increase the energy level of the steam, while convective superheaters rely on the transfer of additional energy from the flue gases to the steam.
Economizers. In many boilers, the flue gases still have useful amounts of energy even after they have passed through the boiler. In many of these applications, economizers provide effective methods of increasing boiler efficiency by transferring the heat of the flue gases to incoming feedwater. There are two principal types of economizers: noncondensing and condensing. Non-condensing economizers are usually air-to-water heat exchangers. Because these economizers are not designed to handle flue gas condensation, noncondensing economizers must be operated at temperatures that are reasonably above the dew points of the flue gas components. The dew point of the flue gases depends largely on the amount of water in the gas, which, in turn, is related to the amount of hydrogen in the fuel. For example, to avoid condensation in the exhaust gases produced by burning natural gas, the exhaust gas temperature should typically be kept above 250°F. Condensing economizers are designed to allow condensation of the exhaust gas components. Due to latent heat recovery, these economizers typically extract more energy than do noncondensing economizers and are used in several industries. Often, special materials are required, such as specialty stainless steels.
Combustion air preheaters. Combustion air preheaters are similar to economizers in that they transfer energy from the flue gases back into the system. In these devices, however, the energy is transferred to the incoming combustion air. The efficiency benefit is roughly 1% for every 40°F increase in the combustion air temperature.6
Boiler Insulation
The walls and combustion regions of boilers are typically lined with insulating materials to reduce energy loss and to prevent leakage. There are several types of boiler insulating materials, including brick, refractory, insulation, and lagging. The selection and design of boiler insulating materials depends largely on the age and design of the boiler. Since the insulating lining is exposed to high temperatures and is subject to degradation, it should be periodically inspected and repaired when necessary. New materials technologies allow efficient insulation in tight spaces previously impossible to insulate.
Boiler Control System
Boiler control systems are designed to protect the boiler and to ensure proper boiler operation. These systems include the combustion control system, flame safeguard, water level control, and fuel control.
Combustion control system. The combustion control system regulates the fuel and air mixture to achieve safe and efficient combustion and maintains proper steam system pressure. Control systems have varying levels of sophistication. Simple systems use a fixed linkage between the fuel-regulating valve and the combustion air damper. This is called single point positioning. A change in steam pressure makes a proportional change in the combustion air and fuel. Modern systems rely on signals from digital transmitters to determine independent fuel valve and air damper positions, and it typically provides alarms for out-of-specification conditions for investigation and remedy. This is called a full monitoring system.
Burner flame safeguard system. A flame safeguard system is an arrangement of flame detection systems, interlocks, and relays which will sense the presence of a proper flame in a furnace and cause fuel to be shut off if a hazardous condition develops. Modern combustion sys-tems are closely interlocked with flame safeguard systems and also pressure-limit switches, low-water level cutoffs, and other safety controls that will stop the energy input to a boiler when an unsafe condition develops. The flame safeguard system senses the presence of a good flame or proper combustion and programs the operation of a burner system so that motors, blowers, ignition, and fuel valves are energized only when they are needed and then in proper sequence.
Safety shut-off valve. Safety shut-off valves isolate the fuel supply to the boiler in response to certain conditions such as low or high gas pressure or satisfied load demand. The type of safety shutoff valves and the settings are often determined by code or insurance requirements.
Water-level control. The boiler water-level control system ensures a safe water level in the boiler. Typically, the control system provides a signal to the feedwater control valve to regulate the feed rate. Simple water-level control systems that only sense water level are single-element systems. More complex systems incorporate additional data such as steam flow rate (dual-element system) and feedwater flow (triple-element system) and will provide better water level control during abrupt load changes.
Safety valve. The safety valve is the most important valve on the boiler and keeps the boiler from exceeding its maximum allowable working pressure (MAWP).
Steam-pressure control. Steam-pressure controls regulate the combustion equipment to maintain a constant pressure in the steam header. As the pressure rises above or falls below the pressure setting, the control adjusts the burner firing rate to bring the pressure back to the setpoint.
Nonreturn valve. The nonreturn valve is a combination shutoff and check valve that allows steam out of the boiler, but prevents backflow from the steam header in the event the boiler pressure drops below that of the header. The valve is opened only when the pressure inside the boiler rises slightly above the steam header pressure.
Steam flow meter. Steam flow meters are helpful in evaluating the performance of the system and can provide useful data in assessing boiler performance, calculating boiler efficiency, and tracking the amount of steam required by the system. In some systems, steam flow meters provide a measurement signal for the boiler control system. Additionally, steam flow meters can be useful in benchmarking efforts.
There are three basic types of steam flow meters: differential pressure (DP), vortex, and Coriolis. Differential pressure flow meters rely on the change in pressure as steam flows by an element such as a nozzle, orifice, or venturi. This pressure difference provides an indication of flow velocity, which, in turn, can be used to determine the flow rate. Vortex flowmeters rely on the principal that flow past an element creates vortices that have frequencies that correspond to the flow velocity. Coriolis flowmeters rely on tubes placed in the steam flow path that twist according to the velocity of the flow.
Boiler Feedwater System
The boiler feedwater system supplies water to the boiler. Sources of feedwater include returning condensate and makeup water. Feedwater is typically stored in a collect-ing tank to ensure that a steady supply of heated water is available to the boiler regardless of momentary fluctuations in availability of condensate return or fresh water supply pressure.
Feedwater flow control valve. A modulating feedwater flow control valve moves up or down in response to the water level transmitter(s). On smaller firetube boilers, it is not uncommon for the feedwater valve to operate in a closed or open position, depending on the water level transmitter signal. In modern industrial boilers, the con-trols may also account for significant variations in steam demand.
Softener. Softeners remove hardness minerals, such as calcium, magnesium, and iron, from a water supply. The presence of hardness in boiler water leads to many problems, including scale buildup and foaming, which reduce boiler efficiency and can cause tube failure. Softeners reduce this problem through an ion exchange process. As the hard water passes through a chamber filled with resin, an exchange occurs that removes hardness minerals from the water. The sodium that replaces the hardness minerals has a higher solubility in water and generally will not form scale.
Pretreatment equipment. Pretreatment equipment improves the quality of the incoming water so that it may be used in the boiler without excessive scaling or foaming, which can reduce boiler efficiency and cause tube failure. Pretreatment equipment includes, but is not limited to, clarifiers, filters, softeners, dealkalizers, decarbonators, reverse osmosis (RO) units, and demineralizers.
Deaerator, deaerating heater, and atmospheric deaerator. The presence of oxygen in the boiler system can be a significant problem because of its corrosivity at high temperatures. Deaerators and deaerating heaters use heat, typically steam, to reduce the oxygen content in water. Deaerators and deaerating heaters are typically pressurized tanks that raise the water temperature to the point of saturation. They also break the incoming water into either fine droplets or thin sheets to facilitate the removal of oxygen and other noncondensable gases. Depending on the design, the feedwater oxygen content can be reduced to levels ranging from 7 to 40 parts per billion (ppb).
Atmospheric deaerators are typically found in smaller, lower-pressure boiler systems. They operate at atmospheric pressure, so the maximum operating temperature is 212°F. Most will operate at temperatures lower than this. Atmospheric deaerators cannot achieve the same level of oxygen removal as deaerators and deaerating heaters, typically providing water with oxygen levels of 0.5 to 1 part per million (ppm).
In applications that require lower oxygen levels than achievable with a deaerator, deaerating heater, or open feedwater heater, a chemical agent, known as an oxygen scavenger, can be used to remove more oxygen. In most systems, an oxygen scavenger is part of the system’s water treatment program.
Feedwater pump. Feedwater pumps transfer water from the deaerator to the boiler. Feedwater pumps are driven by electric motors or by steam turbines. In a modulating feedwater system, the feedwater pumps run constantly as opposed to an on/off operation in relatively small boilers.
Collecting/Storage tank. The return of condensate is often erratic due to changing steam requirements by the end uses. The condensate is usually returned to a condensate receiver or directly to the deaerator if the system does not have a receiver. Pretreated water may also be stored in a tank prior to use. This provides the boiler system with additional water capacity in case the pretreatment equipment malfunctions. The condensate and pretreated water, or makeup, are transferred from the storage tanks to the deaerator before being sent to the boiler.
Boiler Combustion Air System
The combustion air system supplies the oxygen necessary for the combustion reaction. To provide enough air for the amount of fuel used in industrial boilers, fans are typically required. Dampers, inlet valves, or variable speed drives typically control the amount of air allowed into the boiler.
Forced draft fan. A forced draft fan is located at the inlet of a boiler and pushes ambient air into the burner region, ensuring that adequate air is delivered to the combustion process. These fans either pull air directly from the boiler room or connect to a duct system that allows outside air to be drawn into the boiler.
Induced draft fan. Induced draft fans are located on the outlet gas side of the boiler and pull flue gases out. The induced draft fan creates a slightly negative furnace pressure that is controlled by outlet dampers on the boiler. In some systems where a bag house, mechanical collector, or precipitator is involved, special considerations should be given in sizing and selection of this fan.
Damper. Dampers control the amount of air allowed into and out of a combustion chamber. Dampers, in combination with fuel regulating devices, are positioned by the combustion control system to achieve certain fuel-to-air ratios. Dampers on the boiler outlet are used to regulate the negative furnace draft.
Boiler Fuel System
There are many different types of fuels used in boilers, requiring several different types of fuel handling systems. Biomass fuels provide emission options that are carbon neutral. Fossil fuels such as coal, oil, and gas are most commonly used. Waste fuels are used in many industries, particularly the forest products, petroleum refining, and chemical manufacturing industries where there is an avail-able supply of waste products such as bark, wood chips, black liquor, and refinery gas.
Fuel regulating valve. In gaseous and liquid fuels, regulating valves control the fuel delivered to the boiler. In many systems, these valves can be quickly shut in response to an operating problem.
Fuel. The fuel types that are commonly used in boilers include natural gas, coal, propane, fuel oils, and waste fuels (for example, black liquor, bark, and refinery gas). Fuel type significantly affects boiler operation, including efficiency, emissions, and operating cost. In 2005, almost 78% of boiler units and 56% of industrial boiler capacity were identified as natural gas-fired. Some industries use by-product fuel in a large portion of their boiler capacity, including paper (48%), refining (49%), and primary metals (42%). Coal, oil, and wood are important fuels in certain industries and regions, but fuel a small fraction of the boiler capacity on a national basis. More detail is available in the reference below.
Fuel flow meter. Fuel meters measure the amount of fuel delivered to a boiler. Fuel meters provide essential data in determining boiler efficiency. Since fuel flow meters measure volume or mass of fuel, it is important to know the energy content of the fuel when determining boiler efficiency.
Burner. Burners combine the fuel and air to initiate combustion. There are many different types of burners due to the many different types of fuels. Additionally, burners have different performance characteristics and control requirements. Some burners are on/off, while others allow precise setting of the fuel:air mixture over a range of conditions. Some burners can fire different types of fuel, allowing boiler operation to continue despite the loss of one fuel supply.
Boiler Blowdown System
The boiler blowdown system includes the valves and the controls for the continuous blowdown and bottom blow-down services. Continuous blowdown removes a specific amount of boiler water (often measured in terms of percentage of feedwater flow) in order to maintain a desired level of total dissolved solids in the boiler. Setting the flow for the continuous blowdown is typically done in conjunction with the water treatment program. Some continuous blowdown systems rely on the input of sensors that detect the level of dissolved solids in the boiler water.
The bottom blowdown is performed to remove particulates and sludge from the bottom of the boiler. Bottom blow-downs are periodic and are typically performed a certain number of times per shift or according to a set schedule. In some systems, bottom blowdowns are controlled by an automatic timer. Bottom blowdown should never be permitted unless it is recommended by the boiler manufacturer. This is because in higher pressure boilers, especially those above 700 pounds psig, bottom blowdown may cause water starvation in some portions of the boiler circuit.
Boiler blowdown heat exchangers and flash tank. The continuous blowdown water has the same temperature and pressure as the boiler water. Before this high energy water is discharged into the environment, it is often sent to a heat exchanger and flash tank. Flash tanks permit the recovery of low-pressure flash steam, which can be used in deaeration or process heating. They also permit the use of a smaller heat exchanger than would be required without the flash tank. Blowdown heat exchangers are most often used to preheat boiler makeup water.
Performance Improvement Opportunities
Overview
Several important factors should be considered when industrial facilities seek to improve steam system per-formance and to lower operating costs. Improving steam system performance requires assessing the entire system, identifying opportunities, and selecting and implementing the most feasible projects. In turn, this requires a systems approach. Similarly, proper selection of the best projects requires quantifying the benefits and costs of each project. Successful implementation of these projects requires the participation of all system stakeholders including produc-tion, maintenance, and management. Generally, obtaining management participation requires communication of the analyses in economic terms. To address these consider-ations, this section of the course discusses:
- The systems approach
- Common performance improvement opportunities
- Resources that can help identify and assess opportunities
- The economics related to steam system improvements.
Energy Efficiency Statistics
The latest statistics for energy efficiency participation can be found at www.eia.gov in the Manufacturing Energy Consumption data, Consumption and Efficiency section under the Sources & Uses tab.
Systems Approach
Because of the many industrial uses for steam, there are wide ranges of steam system sizes, configurations, end-use applications, and operating practices. As a result, there are many different ways to improve steam system performance and identify improvement opportunities. In general, performance is most effectively optimized when a systems approach is used.
A systems approach analyzes both the supply and demand sides of the system and how they interact, essentially shifting the focus from individual components to total system performance. Often, operators are so focused on the immediate demands of the equipment that they overlook the broader issue of how system parameters affect the equipment. Similarly, a common engineering approach is to break a system down into its basic components or modules, optimize the selection or the design of these components, and then assemble these components to form the system. An advantage to this approach is that it simplifies problems. However, a disadvantage is that it often over-looks the interaction of these components. In contrast, a systems approach evaluates the entire system to determine how the end-use requirements can be most effectively and efficiently served.
A systems approach also recognizes that system efficiency, reliability, and performance are closely related. For example, an efficiency loss such as heat loss across uninsulated pipe surfaces reduces energy available to the end uses and requires boilers to work harder to meet a given demand. Often, energy losses create additional system stresses that accelerate wear and that can create loads for which the system was not originally designed.
Common performance improvement opportunities for the generation, distribution, and recovery areas of a steam system are listed in Table 2.
Strategic, Performance Improvement Opportunities—Management-Based Systems
Systems, Standards, and Certification
AMO works with U.S. industry to support the development of energy management standards and a related certification program anchored to a viable business case. Similar to environmental or quality management systems, changing how energy is managed has resulted in substantial energy performance improvements.
Visit www.eere.energy.gov/energymanagement/ to learn how the various standards are related, as well as information on a web-based toolkit that organizations can use to implement an energy management system consistent with ISO 50001 standards and other existing management standards.
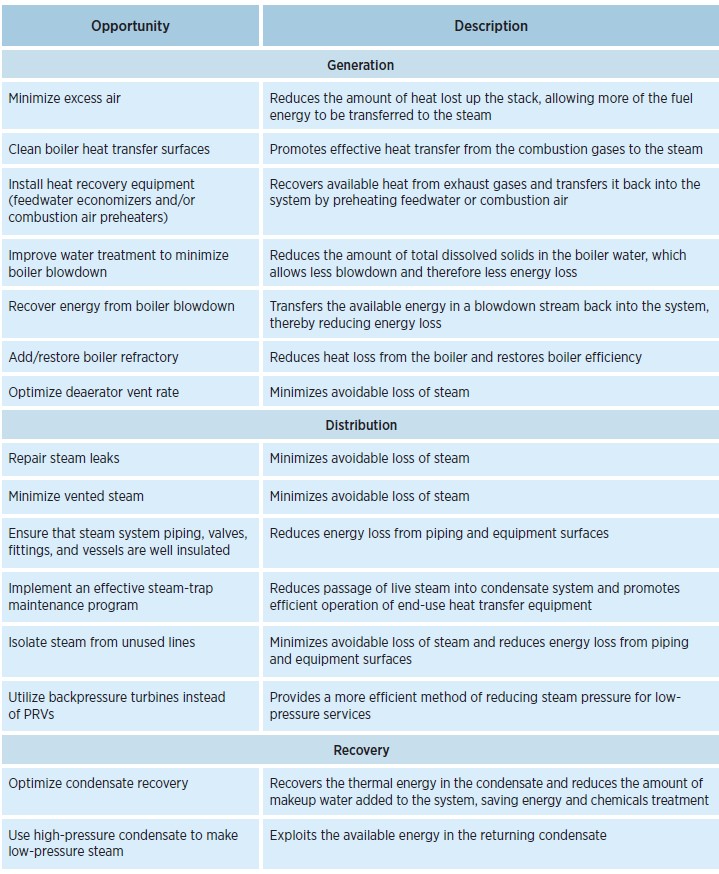
Management Standard – ISO 50001—Plant-Level Energy Management
The energy management standard, ISO 50001, provides a method for integrating continuous improvement in energy efficiency into existing management systems. ISO 50001 creates a framework for organizations to manage energy and improve profitability. The forthcoming industry-designed American National Standards Institute (ANSI)-accredited plant-certification program—known as Superior Energy Performance—will include provisions for conforming to the ISO 50001 energy management standard, as well as achieving annual certification by reaching a defined level of annual, energy-intensity performance improvement.
Superior Energy Performance
Superior Energy Performance (SEP) facilities continuously manage their energy consumption, reduce energy intensity, and validate savings using qualified, independent, measurement and verification entities.
Visit www.superiorenergyperformance.net for details.
Proven Results. Several facilities have piloted the approach in demonstration projects prior to the launch of Superior Energy Performance. These facilities demonstrated impressive results that are nearly twice the efficiency gains of business as usual. SEP has piloted four Texas plants: Cook Composites and Polymers (Houston); Freescale Semiconductor, Inc. (Oak Hill); Owens Corning (Waxahachie); and Union Carbide, a subsidiary of Dow Chemical Company (Texas City). The pilot sites provided substantial input into the design of the program, as did other end users on the U.S. Council for Energy-Efficient Manufacturing. The four pilot sites saw verified energy performance improvements from 6.5% to more than 15% over a 2- to 3-year period.
Four pilot sites verified energy performance improvements from 6.5% to more than 15% in a 2- to 3-year period.
Certification: Documenting Results. A central element of SEP is implementation of the ISO 50001 standard, with additional requirements to achieve and document energy intensity improvements. SEP is designed to encourage participation among facilities of all sizes and all levels of experience in managing energy. The program offers flexibility depending on the degree of data validation desired by an organization or facility. Verification bodies will undertake verification for the SEP program and will be accredited by ANSI. A series of complementary professional credentialing programs train and qualify professionals to assist and audit facilities.
Third-party validation of continuous improvement should be beneficial to organizations in many ways, including improving the chances of securing financing for energy efficiency projects.
Supporting Standards for Steam Assessments. The Association for Mechanical Engineers (ASME) provides a standard that supports industrial steam assessment protocols.
ASME Sidebar PART 1 of 3
The Standard ASME EA-3 – 2009, Energy Assessment for Steam Systems contains detailed sections, including:
Table of Contents
- – Scope and Introduction
- – Definitions
- – References
- – Organizing the Assessment 5 – Conducting the Assessment 6 – Assessment Data Analysis
- – Report and Documentation
ASME Sidebar PART 2 of 3
Abstracted portions from several sections follow:
“4.2 Facility Management Support – Facility management support is essential for the successful outcome of the assessment. Facility management shall understand and support the purpose of the assessment. …”
“5.1 Overall Assessment Method – The overall method to be used in assessing the steam system is a sequential screening process as shown in Fig. 1. This investigation process shall evaluate the operating characteristics of the individual components, subsystems, processes, and the system as a whole …”
“5.4 Target Areas for Assessment – An assessment includes evaluation of the steam sources …, distribution, end use, and condensate recovery. Assessment activities shall focus on quantification of energy losses and the identification …”
“5.7.1 Temperature Measurements … (a) Boiler makeup water … (i) Steam trap …”
“5.7.2 Pressure Measurements … (c) Condensate return tank … (d) Deaerator …”
“5.7.3 Flow Measurements … a) Boiler fuel … c) Makeup water …”
“5.7.4 Chemical Measurements … (a) Boiler makeup water … (e) Condensate …”
Excerpted with permission from ASME.
The excerpts on pp. 25–26 summarize the standard ASME EA-3-2009, Energy Assessments for Steam Systems.
For more details, visit www.asme.org. (Please note that a complete version of the standard is available for purchase on ASME website.)
System auditors may find the following resources detailing performance improvement options valuable.
ASME has developed several systems-level standards that support the above management-level standards approach. These systems-level standards provide detailed protocols for conducting energy assessments.
Energy Assessment for:
- ASME EA-1-2009—Process Heating Systems
- ASME EA-2-2009—Pumping Systems
- ASME EA-3-2009—Steam Systems
- ASME EA-4-2010—Compressed Air Systems
Guidance documents providing specific examples and information related to each section of the above ASME Standards are also available.
ASME EA-3 – 2009 – Energy Assessment for Steam Systems ISBN: 9780791832806
“This standard sets the requirements for conducting and reporting the results of a steam system energy assessment that considers the entire system, from energy inputs to the work performed as the result of these inputs. An assessment meeting this standard shall be sufficiently comprehensive to identify the major opportunities for improving the overall energy performance of the steam system. This standard is designed to be applied primarily at industrial facilities, but most of the specified procedures can be used in other facilities such as those in the institutional and commercial sectors.”
ASME Sidebar PART 3 of 3
Similarly The guidance to the standard, ASME EA-3G – 2010, Guidance for ASME EA-3, Energy Assessment for Steam Systems contains detailed information related to the standard.
Abstracted portions from several sections follow:
“ 4.2 Facility Management Support
Completing an assessment in a large and complex facility can over-extend resources. As a result, it may be appropriate to divide the assessment of a large facility into subsystems with each subsystem being composed of a complete steam system. As an example, a facility equipped with many production units and multiple steam-power generation facilities could choose to complete an assessment on one steam-power generation facility and a selection of production units. Significant care must be given …”
“ 5.1 Overall Assessment Method – General Methodology
Every complete steam system assessment will target the entire steam system (generation, distribution, end-use and recovery). The goal of the evaluation process is to establish the path forward for each aspect of the steam system. The possible ranges of the path forward are very broad. One end of the range would be to maintain the current operating conditions because the system components in question are being operated in an excellent manner and there is minimal incentive to modify operations. Another point within the range could be to …”
“Again, the methodology used for steam system assessments should involve investigation of every aspect of the steam system. In a comprehensive examination every area is targeted and an expert evaluation including a path forward is prescribed. …”
“5.7.1 Temperature Measurements
Temperature measurements are critical to many analyses required in steam system assessments. Temperature is a primary indicator of the energy level of many streams. Temperature is also instrumental in analyzing the condition of insulation covering various equipment components. Commonly temperature is measured with a thermocouple, Resistance Temperature Device (RTD), fluid-in-glass thermometer, or other direct contact thermometer. These devices can be inserted directly in the fluid stream to get a temperature measurement—this is often the case for near atmospheric pressure fluid streams (such as boiler flue gas).”
“5.7.4 Chemical Measurements
Chemical measurements are primarily necessary in the evaluation of the performance of the boiler. Flue gas chemical composition is a critical analysis in the determination of the boiler stack loss. Often the flue gas chemicals are control parameters for the boiler, for example, combustion zone oxygen control and environmental emissions. The most common flue gas chemical components measured are oxygen … and particulate matter.”
Common Performance Improvement Opportunities
Several steam system improvement opportunities are com-mon to many industrial facilities. These opportunities can be categorized according to the part of the system in which they are implemented. Common performance opportunities for the generation, distribution, and recovery areas of a steam system are listed in the Oak Ridge National Laboratory Report from Save Energy Now Assessments in recent steam system assessments.
End-Use Improvement Opportunities
There are many ways to optimize steam system energy use depending on the process and the equipment. Specific end uses within energy intensive industries and related steam systems can be found in Table 1 of the End Use section. In some cases, equipment can be installed to make the process more efficient; for example, multiple-stage dryers are often more efficient than single-stage dryers. However, in general, optimizing the efficiency of steam-supplied end uses requires detailed knowledge and experience and a case-by-case assessment. Many of these same energy sav-ings opportunities were identified as practical options at specific industrial plants that received steam assessments. These assessments were conducted by AMO for large industrial energy-consuming plants from 2006 through 2009. Please visit http://info.ornl.gov/sites/publications/files/Pub25191.pdf ORNL/TM-2010/146 for detailed steam assessments results and to learn how your organization can achieve significant energy savings after implementing recommendations from a steam assessment.
Steam System Scoping Tool
- Introduction—provides instructions on how to use the guide and what is indicated by the results.
- Basic data—prompts the user to answer general questions such as the amount of fuel used, amount of steam generated, and other general system data.
- System profiling—assesses how the user tracks steam costs, benchmarks steam use, and measures important general operating parameters.
- Operating practices of the total system—queries the user regarding practices such as trap maintenance, water treatment, insulation condition, leak repair, and general equipment inspection.
- Operating practices of the boiler plant—queries the user on boiler efficiency, heat recovery equipment, steam quality, and general boiler operation.
- Operating practices of the distribution, end-use, and recovery portions of the steam system—queries the user about the use of pressure reducing valves, condensate recovery, and the use of condensate to generate low-pressure steam.
- Summary sheet—provides scores based on user responses.
Steam System Survey Guide
The Steam System Survey Guide is a reference document that is intended for use by plant energy managers and sys-tem operations personnel. The guide provides a technical basis for identifying and assessing many potential steam system improvement opportunities. Although several of these opportunities can be identified directly with the survey guide, others require more sophisticated measurements and data gathering methods.
The Scoping Tool and the Survey Guide are complementary. The scoping tool allows a user to determine how well the system is performing and is also useful in tracking the effectiveness of system improvements. The survey guide provides a more quantitative description of the system operation and how to quantify some of the potential steam system improvement opportunities. The survey guide includes a Call to Action section in the chapters of Steam System Profiling, Identifying Steam System Properties, Boiler Efficiency, Effectiveness of Resource Utilization, and Distribution System Losses. These tools are available at: manufacturing.energy.gov.
This website also offers an online publications library with links to other resources that can assist end users in improving the performance and efficiency of their energy-intensive utility systems.
Steam System Assessment Tool
The Steam System Assessment Tool allows users to assess potential savings from individualized steam system improvements. Users may input data about the condition of their plant and the Steam System Assessment Tool generates models of various improvement scenarios. Results detail the energy, cost, and emissions savings that a variety of improvements could achieve.
The tool contains all the key features of typical steam systems—boilers, backpressure turbines, condensing turbines, deaerators, letdowns, flash vessels, and feedwater heat exchangers. The model analyzes boiler efficiency, boiler blowdown, cogeneration, steam cost, condensate recovery, heat recovery, vent steam, insulation efficiency, alternative fuels, backpressure turbines, steam traps, steam quality, and steam leaks.
3E Plus Insulation Appraisal Software
Because insulation is used in every steam system, its restoration, replacement, or installation are common improvement opportunities. A lack of awareness regard-ing the energy losses and the associated costs often results in a low prioritization of restoring or properly installing insulation on steam system surfaces. As a result, a software program known as 3E Plus was developed by the North American Insulation Manufacturers Association (NAIMA). The program increases awareness among steam system operations and management personnel of the benefits of insulation and assists these stakeholders in assessing insulation opportunities.
3E Plus assists the user in assessing important insulation project factors such as energy savings, installation cost, and payback period for various insulation materials and thicknesses. Users of 3E Plus can estimate energy losses from uninsulated surfaces, as well as potential savings from various insulation options.
The program has general data for insulation costs by type and can analyze insulation cross-sections that use several different insulation types. It also accounts for labor rates and productivity by region, estimating how difficult the installation process will be based on general piping characteristics. Users can quickly determine the economic feasibility of various insulation thicknesses. Since the program also allows the user to evaluate various combinations of insulation types, 3E Plus can help the user optimize the material thicknesses within an insulation system. Download 3E Plus at manufacturing.energy.gov.
NIA’s Insulation Energy Appraisal Program (IEAP)
The National Insulation Association (NIA), has developed a training program that offers certification to professionals who conduct insulation appraisals or specify insulation requirements. This program is intended to provide credibility to insulation professionals and to increase consistency of the message that is presented to clients. This program has four key components:
- Awareness Building—an important way to increase awareness of the potential cost savings from insulation projects is to effectively promote insulation appraisal as a professional service.
- Information Gathering—determining the parts of
the system that have the most attractive insulation improvement opportunities usually requires input from the plant personnel. Improving the interview techniques of insulation professionals can increase the usefulness of these assessments. - 3E Plus—the 3E Plus program is an important tool for insulation professionals and specifying engineers. Learning to effectively use this tool can improve the quality of the assessment findings, presentation of recommendations, and cost-effective specification of new insulation.
- Reporting—accurately and effectively reporting the results of an insulation assessment can significantly increase the probability that the recommendations will be implemented.
Information about IEAP can be obtained at
www.insulation.org or www.pipeinsulation.org.
Steam Tip Sheets
Some improvement opportunities are available to many different systems. To increase industry awareness of several fundamental improvement opportunities, AMO has developed steam tip sheets.
These steam tip sheets provide concise descriptions of common improvement opportunities. Because AMO continues to develop and identify energy improvement pro-grams, additional tip sheets are expected.
Steam System Training
Online Self-Guided Training
This online course covers the operation of typical steam systems and discusses methods of system efficiency improvement. The training is designed for plant person-nel, such as energy managers, steam system supervisors, engineers, and equipment operators, who have steam system responsibilities in industrial and institutional plants. The course covers three key areas of potential system improvement:
- Steam Generation Efficiency
- Resource Utilization Effectiveness
- Steam Distribution System Losses
The course introduces the Steam System Scoping Tool and the Steam System Assessment Tool, both developed by AMO and uses the Steam System Survey Guide as a technical reference. The training also introduces the 3E Plus insulation appraisal software and a course example is presented that uses this software.
Steam Tool Specialist Training
Industry professionals can earn recognition as specialists in the use of the AMO Steam Tools. DOE offers an in-depth, multiday training for steam system specialists, including two days of classroom instruction and a written exam. Participants who complete the training and pass the written exam and maintain certification are recognized by DOE as Steam Tool Specialists, and are listed on the AMO website. Specialists can assist industrial customers in using the AMO Steam Tools to evaluate their steam systems.
The AMO Steam System Specialist training is primarily designed for steam specialists who are interested in becoming proficient in using the AMO Steam Tools. To successfully complete the Specialist Training course, a participant must understand the full suite of AMO Steam tools, including:
Steam System Survey Guide
The Steam System Survey Guide provides technical information for steam system operational personnel and plant energy managers on major areas where steam systems can be improved. The guide outlines calculations that can be performed to quantify steam system improvement opportunities.
Steam System Scoping Tool
- Accurate collection and input of data for use with the tool
- Appropriate utilization of the software
- Interpretation of the software results.
Steam System Assessment Tool
- Accurate collection and input of data for use with the tool
- Development of representative system models
- SSAT limitations
- Individual project identification
- Development of practical methods to accomplish the appropriate projects.
3E Plus Training
- Accurate data gathering for basic insulation-related evaluations
- Development of evaluation techniques related to common insulation evaluations.
Class participants receive a prerequisite test/study guide before attending the class. This study guide provides an indicator of the skill levels necessary to successfully com-plete the training. Completion of this study guide is highly recommended, but is not a requirement to take the training. Completion of AMO’s Steam End-User training is also recommended prior to participating in the Specialist training because it covers the majority of the prerequisites.
Learn more about the Steam System training at: manufacturing.energy.gov.
Financing Steam System Improvements
Industrial facility managers often must convince corporate decision-makers that an investment in steam efficiency is worth the effort. Often, communicating this message can be more challenging than the technical implementation of the concept. The corporate audience will respond more readily to a message of economic impact than a discussion of Btu, pounds of steam, and efficiency ratios. By adopting a financial approach, the facility manager relates steam efficiency to corporate goals. Collaboration with financial staff can yield the kind of proposal that is needed to convince corporate officers who have the final word about capital investments such as steam system upgrades.
Before developing recommendations for how to justify steam improvement projects, it is useful to understand the world as the corporate office usually sees it.
Understanding Corporate Priorities
Corporate officers are held accountable to a chief executive, a board of directors, and an owner (or shareholders, if the firm is publicly held). It is the job of these officers to create and grow the equity value of the firm. The corporation’s industrial facilities do so by generating revenue that exceeds the cost of owning and operating the facility itself. Plant equipment—including steam system components—is an asset that must generate an economic return. The annual earnings attributable to the sale of goods produced by these assets, divided by the value of the plant assets themselves, describe the rate of return on assets.
This is a key measure by which corporate decision-makers are held accountable. Financial officers seek investments that are most certain to demonstrate a favorable return on assets. When faced with multiple investment opportunities, the officers will favor those options that lead to both the largest and fastest returns.
This corporate attitude may impose (sometimes unpleasant) priorities on the facility manager: assure reliability in production, avoid unwanted surprises by sticking with familiar technology and practices, and contribute to cost control today by cutting a few corners in maintenance and upkeep. This may result in industrial decision-makers concluding that steam efficiency is a “luxury” they cannot afford. Fortunately, the story does not end here. What follows is a discussion of ways that industrial steam efficiency can save money and contribute to corporate goals while effectively reducing energy consumption and cutting noxious combustion emissions.
Measuring the Dollar Impact of Steam Efficiency
Steam efficiency improvements can move to the top of the list of corporate priorities if the proposals respond to distinct corporate needs. Corporate challenges are many and varied, which in turn open up more opportunities to “sell” steam efficiency as a solution. Steam systems offer many opportunities for improvement; the particulars are shared elsewhere in this course. Once the selections are made, the task is one of communicating the proposals in corporate financial language.
The first step is to identify and enumerate the total dollar impact of a steam efficiency measure. One framework for this is known as “life-cycle cost analysis.” These analyses capture the sum total of expenses and benefits associated with an investment. The result—a net gain or loss on balance—can be compared to other investment options or to the anticipated outcome if no investment is made. As a comprehensive accounting of an investment option, the life-cycle cost analysis for a steam efficiency measure would include projections of:
- Search and selection costs for seeking an engineering implementation firm
- Initial capital costs, including asset purchase, installation, and costs of borrowing
- Maintenance costs
- Supply and consumable costs
- Energy costs over the economic life of the implementation
- Depreciation and tax impacts
- Scrap value or cost of disposal at the end of the equipment’s economic life
- Impacts on production such as product quality and downtime.
One revelation that typically emerges from this exercise is that fuel costs may represent as much as 96% of life-cycle costs, while the initial capital outlay is only 3%, and maintenance a mere 1%. These findings may be true for boilers with a 20-year life operating at high rates of capacity utilization. Clearly, any measure that reduces fuel consumption (while not impacting reliability and productivity) will certainly yield positive financial impacts for the company.
Financing Steam Efficiency Improvements
As with any corporate investment, there are many ways to measure the financial impact of steam efficiency investments. Some methods are more complex than others are, and proposals may use several analytical methods side-by-side. The choice of analyses used will depend on the sophistication of the presenter and the audience.
A simple and widely used measure of project economics is the payback period. This is defined as the period of time required for a project to “break even.” It is the time needed for the net benefits of an investment to accrue to the point where they equal the cost of the initial outlay. For a project that returns benefits in consistent, annual increments, the simple payback equals the initial investment divided by the annual benefit. Simple payback does not take into account the time value of money; in other words, it makes no distinction between a dollar earned today versus a dollar of future (and therefore uncertain) earnings. Still, the measure is easy to use and understand and many companies use simple payback for a quick “go/no-go” decision on a project. Five important factors to remember when calculating a simple payback:
- It is an approximation, not an exact economic analysis
- All benefits are measured without considering their timing
- All economic consequences beyond the payback are ignored
- Payback calculations will not always find the best solution (for the two reasons immediately above) when choosing among several project options
- Payback does not consider the time value of money or tax consequences.
More sophisticated analyses take into account factors such as discount rates, tax impacts, the cost of capital, etc. One approach involves calculating the net present value of a project, which is defined in the equation below:
Net present value = Present worth of benefits – Present worth of costs
Another commonly used calculation for determining economic feasibility of a project is internal rate of return, which is defined as the discount rate that equates future net benefits (cash) to an initial investment outlay. This discount rate can be compared to the interest rate at which a corporation borrows capital.
Many companies set a threshold (or hurdle) rate for projects, which is the minimum required internal rate of return for a project to be considered viable. Future benefits are discounted at the threshold rate, and the net present worth of the project must be positive in order for the project to be a “go.”
Relating Steam Efficiency to Corporate Priorities
Saving money should be a strong incentive for adopting steam efficiency. Still, that may not be enough for some corporate observers. The facility manager’s case can be strengthened by relating a positive life-cycle cost outcome to specific corporate needs. Some suggestions for interpreting the benefits of fuel cost savings include the following (finance staff can suggest which of these approaches are best for the current corporate climate):
A new source of permanent capital. Reduced fuel expenditures—the direct benefit of steam efficiency—can be thought of as a new source of capital for the corporation. The investment that makes this efficiency possible will yield annual savings each year over the economic life of the improved steam system. Regardless of how the steam efficiency investment is financed—borrowing, retained earnings, or third party financing—the annual savings will be a permanent source of funds as long as the steam efficiency savings are maintained on a continuous basis.
Added shareholder value. Publicly held corporations usually embrace opportunities to enhance shareholder value. Steam efficiency can be an effective way to capture new value. Shareholder value is the product of two variables: annual earnings and the price-to-earnings (P/E) ratio. The P/E ratio describes the corporation’s stock value as the current stock price divided by the most recent annual earnings per share. To take advantage of this measure, the steam efficiency proposal should first identify annual savings (or rather, addition to earnings) that the proposal will generate. Multiplying that earnings increment by the P/E ratio yields the total new shareholder value attributable to the steam efficiency implementation.
Reduced cost of environmental compliance. Facility managers can proactively seek to limit the corporation’s exposure to penalties related to environmental emissions compliance. Steam efficiency, as total-system discipline, leads to better monitoring and control of fuel use. Combustion emissions are directly related to fuel consumption; they rise and fall in tandem. By implementing steam efficiency, the corporation enjoys two benefits: decreased fuel expenditures per unit of production, and fewer incidences of emission-related penalties.
Improved worker comfort and safety. Steam system optimization requires ongoing monitoring and maintenance that yields safety and comfort benefits in addition to fuel savings. The routine involved in system monitoring will usually identify operational abnormalities before they present a danger to plant personnel. Containing these dangers precludes threats to life, health, and property.
Improved reliability and capacity utilization. Another benefit to be derived from steam efficiency is more productive use of steam assets. The efforts required to achieve and maintain energy efficiency will largely contribute to operating efficiency. By ensuring the integrity of steam system assets, the facility manager can promise more reliable plant operations. The flip side, from the corporate perspective, is a greater rate of return on assets employed in the plant.
Call to Action
A proposal for steam efficiency implementation can be made attractive if the facility manager:
- Uses an energy management system that meets the supply chain energy needs of the customer company
- Identifies opportunities for achieving steam efficiency
- Determines the life-cycle cost of attaining each option
- Identifies the option(s) with the greatest net benefits
- Collaborates with financial staff to identify current corporate priorities (for example, added shareholder value, reduction in environmental impact and environmental compliance costs, and improved capacity utilization)
- Generates a proposal that demonstrates how the steam efficiency project’s benefits will directly respond to current corporate needs
- Generates a proposal that identifies incentives avail-able from utilities or states or other sources.
Summary
Increased awareness of the potential improvements in steam system efficiency and performance is an important step toward increasing the competitive capabilities of energy-intensive industries. Some of the useful steam resources and tools developed by AMO have been described in this section. Additional steam resources and tools and where to obtain them are described in the Resources and Tools section.
Steam System Tip Sheets (1-4)
Inspect and Repair Steam Traps
In steam systems that have not been maintained for 3 to 5 years, between 15% to 30% of the installed steam traps may have failed—thus allowing live steam to escape into the condensate return system. In systems with a regularly scheduled maintenance program, leaking traps should account for less than 5% of the trap population. If your steam distribution system includes more than 500 traps, a steam trap survey will probably reveal significant steam losses.
Example
In a plant where the value of steam is $10.00 per thousand pounds ($10.00/1,000 lb), an inspection program indicates that a trap on a 150-pound-per-square-inch-gauge (psig) steam line is stuck open. The trap orifice is 1/8th inch in diameter. The table shows the estimated steam loss as 75.8 pounds per hour (lb/hr). After the failed trap is repaired, annual savings are:

Steam Trap Testing Facts
Steam traps are tested to determine if they are functioning properly and not cold plugging or failing in an open position and allowing live steam to escape into the condensate return system. There are four basic ways to test steam traps: temperature, sound, visual, and electronic.
Recommended Steam Trap Testing Intervals
- High-Pressure (150 psig and above): Weekly to Monthly
- Medium-Pressure (30 to 150 psig): Monthly to Quarterly
- Low-Pressure (below 30 psig): Annually
For additional information on monitoring, download the following sub-metering case studies from the AMO publication library:
- Solutia: Utilizing Sub-Metering to Drive Energy Project Approvals Through Data
- Nissan North America: How Sub-Metering Changed the Way a Plant Does Business
Also refer to the following guidebook on the EERE Federal Energy Management website at www.femp.energy.gov:
- Metering Best Practices: A Guide to Achieving Utility Resource Efficiency, Release 2.0
Insulate Steam Distribution and Condensate Return Lines
Uninsulated steam distribution and condensate return lines are a constant source of wasted energy. The table shows typical heat loss from uninsulated steam distribution lines. Insulation can typically reduce energy losses by 90% and help ensure proper steam pressure at plant equipment. Any surface over 120°F should be insulated, including boiler surfaces, steam and condensate return piping, and fittings.
Insulation frequently becomes damaged or is removed and never replaced during steam system repair. Damaged or wet insulation should be repaired or immediately replaced to avoid compromising the insulating value. Eliminate sources of moisture prior to insulation replacement. Causes of wet insulation include leaking valves, external pipe leaks, tube leaks, or leaks from adjacent equipment. After steam lines are insulated, changes in heat flows can influence other parts of the steam system.

Example
In a plant where the fuel cost is $8.00 per million Btu ($8.00/MMBtu), a survey of the steam system identified 1,120 feet (ft) of bare 1-inch-diameter steam line, and 175 feet of bare 2-inch line, both operating at 150 pounds per square inch gauge
(psig). An additional 250 ft of bare 4-inch-diameter line operating at 15 psig was found. From the table, the quantity of heat lost per year is:
Given a boiler efficiency of 80%, the annual cost savings from installing 90% efficient insulation is:
Insulation Optimization Software Available
The North American Insulation Manufacturers Association has developed a software package (3E Plus) that determines the optimum thickness for a wide variety of insulating materials. Outputs include the simple payback period, surface heat loss, and surface temperature for each specified insulation thickness. 3E Plus is available at no cost on AMO’s website at manufacturing.energy.gov
Use Insulating Jackets
Removable insulating jackets are available for valves, flanges, steam traps, and other fittings. Remember that a 6-inch gate valve may have more than 6 square feet of surface area from which to radiate heat.
Install insulating jackets on steam traps according to steam trap manufacturer’s directions to maintain proper trap operation.
For additional information on removable insulation, refer to the related steam tip sheet #17 Install Removable Insulation on Valves and Fittings in the publication library on the AMO website.
Use Feedwater Economizers for Waste Heat Recovery
A feedwater economizer reduces steam boiler fuel requirements by transferring heat from the flue gas to incoming feedwater. Boiler flue gases are often rejected to the stack at temperatures more than 100°F to 150°F higher than the temperature of the generated steam. Generally, boiler efficiency can be increased by 1% for every 40°F reduction in flue gas temperature. By recovering waste heat, an economizer can often reduce fuel requirements by 5% to 10% and pay for itself in less than 2 years. The table provides examples of the potential for heat recovery.

Example
An 80% efficient boiler generates 45,000 pounds per hour (lb/hr) of 150-pounds-per-square-inch-gauge (psig) steam by burning natural gas. Condensate is returned to the boiler and mixed with makeup water to yield 117°F feedwater. The stack temperature is measured at 500°F. Determine the annual energy savings that will be achieved by installing an economizer given 8,400 hours per year (hr/yr) of boiler operation at a fuel cost of $8.00 per million Btu ($8.00/MMBtu).
From the steam tables, the following enthalpy values are available:
The recoverable heat corresponding to a stack temperature of 500°F and a natural gas-fired boiler load of 50 MMBtu/hr is read from the table (above) as 4.6 MMBtu/hr.
Exhaust Gas Temperature Limits
The lowest temperature to which flue gases can be cooled depends on the type of fuel used: 250°F for natural gas, 300°F for coal and low sulphur content fuel oils, and 350°F for high sulphur fuel oils. These limits are set to prevent condensation and possible corrosion of the stack.
Potential Economizer Applications
A feedwater economizer is appropriate when insufficient heat transfer surface exists within the boiler to remove combustion heat. Boilers that exceed 100 boiler horse-power, operating at pressures exceeding 75 psig or above, and that are significantly loaded all year long are excellent candidates for an economizer retrofit.
For additional information on heat recovery, refer to the factsheet titled Unlock Energy Savings with Waste Heat Recovery in the publication library on the AMO website.
Improve Your Boiler’s Combustion Efficiency
Combustion Efficiency
Operating your boiler with an optimum amount of excess air will minimize heat loss up the stack and improve combustion efficiency. Combustion efficiency is a measure of how effectively the heat content of a fuel is transferred into usable heat. The stack temperature and flue gas oxygen (or carbon dioxide) concentrations are primary indicators of combustion efficiency.
Given complete mixing, a precise or stoichiometric amount of air is required to completely react with a given quantity of fuel. In practice, combustion conditions are never ideal, and additional or “excess” air must be supplied to completely burn the fuel.
The correct amount of excess air is determined from analyzing flue gas oxygen or carbon dioxide concentrations. Inadequate excess air results in unburned combustibles (fuel, soot, smoke, and carbon monoxide), while too much results in heat lost due to the increased flue gas flow—thus lowering the overall boiler fuel-to-steam efficiency. The table relates stack readings to boiler performance.

On well-designed natural gas-fired systems, an excess air level of 10% is attainable. An often-stated rule of thumb is that boiler efficiency can be increased by 1% for each 15% reduction in excess air or 40°F reduction in stack gas temperature.
Example
A boiler operates for 8,000 hours per year and annually consumes 500,000 million Btu (MMBtu) of natural gas while producing 45,000 lb/hour of 150-psig steam. Stack gas measurements indicate an excess air level of 44.9% with a flue gas minus combustion air temperature of 400°F. From the table, the boiler combustion efficiency is 78.2% (E1). Tuning the boiler reduces the excess air to 9.5% with a flue gas minus combustion air temperature of 300°F. The boiler combustion efficiency increases to 83.1% (E2). Assuming a fuel cost of $8.00/MMBtu, the annual savings are:
Flue Gas Analyzers
The percentage of oxygen in the flue gas can be measured by inexpensive gas-absorbing test kits. More expensive (ranging in cost from $500 to $1,000) hand-held, computer-based analyzers display percent oxygen, stack gas temperature, and boiler efficiency. They are a recommended investment for any boiler system with annual fuel costs exceeding $50,000.
Oxygen Trim Systems
When fuel composition is highly variable (such as refinery gas, hog fuel, or multi-fuel boilers), or where steam flows are highly variable, an online oxygen analyzer should be considered. The oxygen “trim” system provides feedback to the burner controls to automatically minimize excess combustion air and optimize the air-to-fuel ratio.
For additional information on monitoring, download the following sub-metering case studies from the AMO publication library:
- Solutia: Utilizing Sub-Metering to Drive Energy Project Approvals Through Data
- Nissan North America: How Sub-Metering Changed the Way a Plant Does Business
Also refer to the following guidebook on the EERE Federal Energy Management website at www.femp.energy.gov:
- Metering Best Practices: A Guide to Achieving Utility Resource Efficiency, Release 2.0
Steam System Tip Sheets (5-8)
Clean Firetube Boiler Waterside Heat Transfer Surfaces
The prevention of scale formation in firetube boilers can result in substantial energy savings. Scale deposits occur when calcium, magnesium, and silica, commonly found in most water supplies, react to form a continuous layer of material on the waterside of the boiler heat exchange tubes.
Scale creates a problem because it typically possesses a thermal conductivity, an order of magnitude less than the corresponding value for bare steel. Even thin layers of scale serve as an effective insulator and retard heat transfer. The result is overheating of boiler tube metal, tube failures, and loss of energy efficiency. Fuel consumption may increase by up to 5% in firetube boilers because of scale. The boilers steam production may be reduced if the firing rate cannot be increased to compensate for the decrease in combustion efficiency. Energy losses as a function of scale thickness and composition are given in the table below.

Example
A firetube boiler annually uses 450,000 million Btu (MMBtu) of fuel while operating for 8,000 hours at its rated capacity of 45,000 pounds per hour (lb/hr) of 150 pounds per square inch gauge (psig) steam. If scale 1/32nd of an inch thick is allowed to form on the boiler tubes, and the scale is of “normal” composition, the table indicates a fuel loss of 2%.The increase in operating costs, assuming energy is priced at $8.00 per million Btu ($8.00/MMBtu), is:
Monitor Flue Gas Temperature
Scale or deposits serve as insulation and reduce the rate of heat transfer across boiler tubes. Increased scale thickness results in a decrease in boiler efficiency because of an increase in flue gas temperature. Trending of stack gas tempera-ture over time—at a constant firing rate and excess oxygen concentration—can provide a good indication of scale buildup. Energy losses caused by increased stack gas temperature are reduced in firetube boilers that are equipped with heat recovery equipment, such as feedwater economizers, condensing economizers, or air preheaters.
Perform Visual Inspections
Visually inspect boiler tubes when the unit is shut down for maintenance. Scale removal can be achieved by mechanical means or acid cleaning. If scale is present, consult with your local water treatment specialist and consider modifying your feedwater treatment or chemical additives schedule.
Return Condensate to the Boiler
When steam transfers its heat in a manufacturing process, heat exchanger, or heating coil, it reverts to a liquid phase called condensate. An attractive method of improving your power plant’s energy efficiency is to increase the condensate return to the boiler.
Returning hot condensate to the boiler makes sense for several reasons. As more condensate is returned, less make-up water is required, saving fuel, makeup water, and chemicals and treatment costs. Less condensate discharged into a sewer system reduces disposal costs. Return of high purity condensate also reduces energy losses due to boiler blowdown. Significant fuel savings occur as most returned condensate is relatively hot (130°F to 225°F), reducing the amount of cold makeup water (50°F to 60°F) that must be heated.
A simple calculation indicates that energy in the condensate can be more than 10% of the total steam energy content of a typical system. The graph shows the heat remaining in the condensate at various condensate temperatures, for a steam system operating at 100 pounds per-square-inch-gauge (psig), with makeup water at 55°F.
Example
Consider a steam system that returns an additional 10,000 pounds per hour (lb/hr) of condensate at 180°F after distribution modifications. Assume this system operates 8,000 hours annually with an average boiler efficiency of 80%, and makeup water temperature of 55°F. The water and sewage costs for the plant are $0.002 per gallon ($/gal), and the water treatment cost is $0.002/gal. The fuel cost is $8.00 per million Btu ($8.00/MMBtu). Assuming a 12% flash steam loss,* calculate overall savings.
Condensate Recovery Produces Savings
A large specialty paper plant reduced its boiler makeup water rate from about 35% of steam production to between 14% and 20% by returning additional condensate. Annual savings added up to more than $300,000.
Minimize Boiler Blowdown
Minimizing your blowdown rate can substantially reduce energy losses, as the temperature of the blown-down liquid is the same as that of the steam generated in the boiler. Minimizing blowdown will also reduce makeup water and chemical treatment costs.
As water evaporates in the boiler steam drum, solids present in the feedwater are left behind. The suspended solids form sludge or sediments in the boiler, which degrades heat transfer. Dissolved solids promote foaming and carryover of boiler water into the steam. To reduce the levels of suspended and total dissolved solids (TDS) to acceptable limits, water is periodically discharged or blown down from the boiler. Mud or bottom blowdown is usually a manual procedure done for a few seconds on intervals of several hours. It is designed to remove suspended solids that settle out of the boiler water and form a heavy sludge. Surface or skimming blowdown
is designed to remove the dissolved solids that concentrate near the liquid surface. Surface blowdown is often a continuous process.
Insufficient blowdown may lead to carryover of boiler water into the steam, or the formation of deposits. Excessive blowdown will waste energy, water, and chemicals. The optimum blowdown rate is determined by various factors including the boiler type, operating pressure, water treatment, and quality of makeup water. Blowdown rates typically range from 4% to 8% of boiler feedwater flow rate, but can be as high as 10% when makeup water has a high solids content.
Example
Assume that the installation of an automatic blowdown control system (see page 2) reduces your blowdown rate from 8% to 6%. This example assumes a continuously operating natural gas-fired, 150-psig, 100,000-pound-per-hour (lb/hr) steam boiler. Assume a makeup water temperature of 60°F, boiler efficiency of 80%, with fuel valued at $8.00 per million Btu ($8.00/MMBtu), and the total water, sewage, and treatment costs at $0.004 per gallon. Calculate the total annual cost savings.
Automatic Blowdown Control Systems
These systems optimize surface blowdown by regulating water volume dis-charged in relation to amount of dissolved solids present. Conductivity, TDS, silica or chlorides concentrations, and/or alkalinity are reliable indicators of salts and other contaminants dissolved in boiler water. A probe provides feedback to a controller driving a modulating blowdown valve. An alternative is proportional control – with the blowdown rate set proportional to the makeup water flow.
Cycles of Concentration
“Cycles of concentration” refers to the accumulation of impurities in the boiler water. If the boiler water contains 10 times the level of impurities in the makeup water, it is said to have 10 cycles of concentration.
References
- “Consensus Operating Practices for Control of Feedwater/Boiler Water Chemistry in Modern Industrial Boilers,” published by the ASME, 1994.
- “Recommended Rules for the Care and Operation of Heating Boilers,” Section VI of the ASME Boiler and Pressure Vessel Code, 1995.
- “Recommended Guidelines for the Care of Power Boilers,” Section VII of the ASME Boiler and Pressure Vessel Code, 1995.
Recover Heat from Boiler Blowdown
Heat can be recovered from boiler blowdown by using a heat exchanger to preheat boiler makeup water. Any boiler with continuous blowdown exceeding 5% of the steam rate is a good candidate for the introduction of blowdown waste heat recovery. Larger energy savings occur with high-pressure boilers. The following table shows the potential for heat recovery from boiler blowdown.
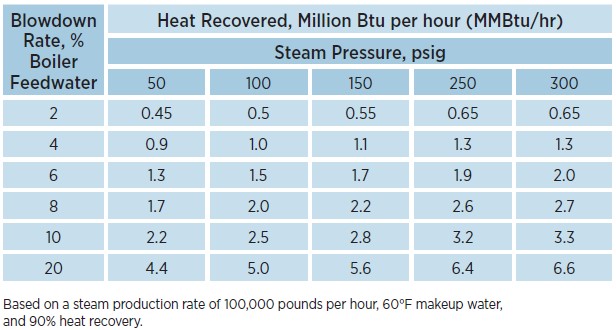
Example
In a plant where the fuel cost is $8.00 per million Btu ($8.00/MMBtu), a continuous blowdown rate of 3,200 pounds per hour (lb/hr) is maintained to avoid the buildup of high concentrations of dissolved solids. What are the annual savings if a makeup water heat exchanger is installed that recovers 90% of the blowdown energy losses? The 80% efficient boiler produces 50,000 pounds per hour (lb/hr) of 150-pounds-per-square-inch-gauge (psig) steam. It operates for 8,000 hours per year. The blowdown ratio is:
From the table, the heat recoverable corresponding to a 6% blowdown ratio with a 150-psig boiler operating pressure is 1.7 MMBtu/hr. Since the table is based on a steam production rate of 100,000 lb/hr, the annual savings for this plant are:
Blowdown Energy Recovery
Blowdown waste heat can be recovered with a heat exchanger, a flash tank, or flash tank in combination with a heat exchanger. Lowering the pressure in a flash tank allows a portion of the blowdown to be converted into low-pressure steam. This low-pressure steam is most typically used in deaerators. Drain water from the flash tank is then routed through a heat exchanger. Cooling the blowdown has the additional advantage of helping to comply with local codes limiting the discharge of high-temperature liquids into the sewer system.
For additional information on heat recovery, refer to the factsheet Unlock Energy Savings with Waste Heat Recovery in the publication library on the AMO website.
Steam System Tip Sheets (9-13)
Use Vapor Recompression to Recover Low-Pressure Waste Steam
Low-pressure steam exhaust from industrial operations such as evaporators or cookers is usually vented to the atmosphere or condensed in a cooling tower. Simultaneously, other plant operations may require intermediate-pressure steam at 20 to 50 pounds per square inch gauge (psig). Instead of letting down high-pressure steam across a throttling valve to meet these needs, low-pressure waste steam can be mechanically compressed or boosted to a higher pressure so that it can be reused.
Vapor recompression relies upon a mechanical compressor or steam jet ejector to increase the temperature of the latent heat in steam to render it usable for process duties. Recompression typically requires only 5% to 10% of the energy required to raise an equivalent amount of steam in a boiler.
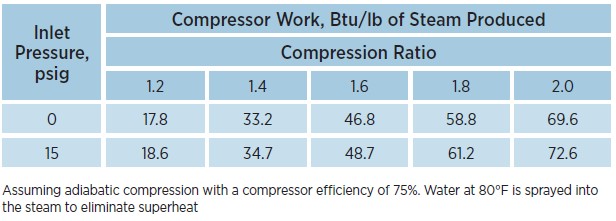
Example
Consider a petrochemical plant that vents 15-psig steam to the atmosphere. At the same time, a process imposes a continuous requirement on the boiler for 5,000 pounds per hour (lb/hr) of 40-pounds-per-square-inch-gauge (psig) steam. If 15-psig waste steam is recompressed to 40 psig by an electrically driven compressor, the compression ratio is:
Interpolating from the table above, the compressor requires 63.5 Btu/lb of delivered steam. Assuming that electricity is priced at $0.06/kWh, the annual cost of driving the compressor is:
If an equivalent quantity of 40-psig steam (enthalpy for saturated steam is 1,176 Btu/lb) were to be supplied by an 80% efficient natural-gas-fired boiler, the steam production costs with fuel priced at $8.00 per million Btu ($8.00/MMBtu) and 70°F feedwater (enthalpy is 38 Btu/lb) are:
Conduct a Pinch Analysis
Based on the actual application, there may be other options to vapor recompression. The industry best practice is to conduct a pinch analysis on the steam system to reveal cost-effective alternatives and optimize steam use by eliminating inefficiencies.
Vapor Recompression Limits
Vapor recompression is limited to applications where the compressor inlet pressure is above atmospheric and the compression ratio is less than two per stage.
System Pressure Boosting
Vapor recompression can be used in steam distribution systems to boost system pressures that have dropped to unacceptably low levels.
Flash High-Pressure Condensate to Regenerate Low-Pressure Steam
Low-pressure process steam requirements are usually met by throttling high-pressure steam, but a portion of the process requirements can be achieved at low cost by flashing high-pressure condensate. Flashing is particularly attractive when it is not economically feasible to return the high-pressure condensate to the boiler. In the table below, the quantity of steam obtained per pound of condensate flashed is given as a function of both condensate and steam pressures.
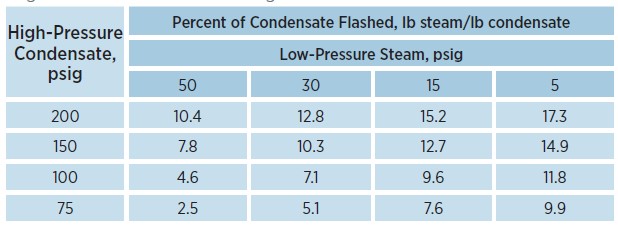
Example
In a plant where the cost of steam is $8.00 per million Btu ($8.00/MMBtu), saturated steam at 150 pounds per-square-inch-gauge (psig) is generated, and a portion of it throttled to supply 30-psig steam. Assuming continuous operation, determine the annual energy savings of producing low-pressure steam by flashing 5,000 pounds per hour (lb/hr) of 150-psig condensate. The average temperature of the boiler makeup water is 70°F.
From the table above, when 150-psig condensate is flashed at 30 psig, 10.3% of the condensate vaporizes.
Proximity Is a Plus
The source of high-pressure condensate should be relatively close to the low-pressure steam header to minimize piping and insulation costs.
Match Availability and Use
The economics of heat recovery projects are most favorable when the waste steam heat content is high and the flow is continuous. Seasonal space heating is not the most desirable end use.
Use a Vent Condenser to Recover Flash Steam Energy
When the pressure of saturated condensate is reduced, a portion of the liquid
“flashes” to low-pressure steam. Depending on the pressures involved, the flash steam contains approximately 10% to 40% of the energy content of the original condensate. In most cases, including condensate receivers and deaerators, the flashing steam is vented and its energy content lost. However, a heat exchanger can be placed in the vent to recover this energy. The following table indicates the energy content of flash steam at atmospheric pressure.

Example
Consider a vent pipe with the following conditions:
A vent condenser could condense the flashed steam, transfer its thermal energy to incoming makeup water, and then return it to the boiler. Energy is recovered in two forms: hotter makeup water and clean, distilled condensate ready for productive use in your operation.
Referring to the table above, the potential energy recovered from the flashed steam is 555 MMBtu, based on 8,760 hours of annual operation. Correct this value for actual operating hours and boiler efficiency:
Distilled Water Recovery
A useful rule of thumb is that every 500 lb/hr of recovered flash steam provides 1 gallon per minute of distilled water.
Materials Considerations
Depending on the specific application, the vent condenser materials can be either all stainless or mild steel shell with copper tubes. For deaerator vent condensing, a stainless steel heat exchanger is recommended to avoid corrosion due to the high concentrations of gases. Mild steel can be used for receiver tank vent condensing.
Use Low-Grade Waste Steam to Power Absorption Chillers
Absorption chillers use heat, instead of mechanical energy, to provide cooling. The mechanical vapor compressor is replaced by a thermal compressor (see figure) that consists of an absorber, a generator, a pump, and a throttling device. The refrigerant vapor from the evaporator is absorbed by a solution mixture in the absorber. This solution is then pumped to the generator where the refrigerant is revaporized using a waste steam heat source. The refrigerant-depleted solution is then returned to the absorber via a throttling device. The two most common refrig-erant/absorbent mixtures used in absorption chillers are water/lithium bromide and ammonia/water.
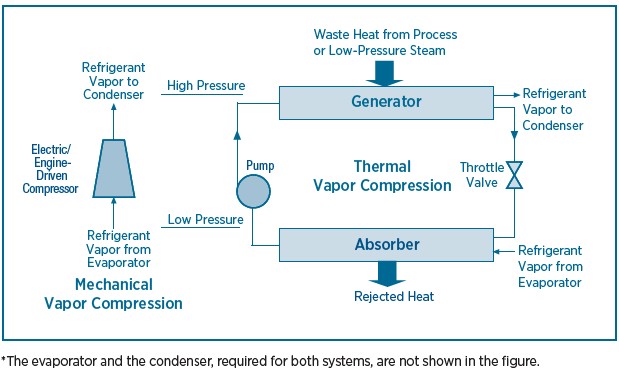
Compared to mechanical chillers, absorption chillers have a low coefficient of performance (COP = chiller load/heat input). Nonetheless, they can substantially reduce operating costs because they are energized by low-grade waste heat, while vapor compression chillers must be motor- or engine-driven.
Low-pressure, steam-driven absorption chillers are available in capacities ranging from 100 to 1,500 tons. Absorption chillers come in two commercially available designs: single-effect and double-effect. Single-effect machines provide a thermal COP of 0.7 and require about 18 pounds of 15-pounds-per-square-inch-gauge (psig) steam per ton-hour of cooling. Double-effect machines are about 40% more efficient, but require a higher grade of thermal input, using about 10 pounds of 100- to 150-psig steam per ton-hour.
Example
In a plant where low-pressure steam is currently being exhausted to the atmo-sphere, a mechanical chiller with a COP of 4.0 is used 4,000 hours per year (hr/yr) to produce an average of 300 tons of refrigeration. The cost of electricity at the plant is $0.06 per kilowatt-hour (kWh).
An absorption unit requiring 5,400 pounds per hour of 15-psig steam could replace the mechanical chiller, providing annual electrical cost savings of:
Steam System Tip Sheets (14-17)
Benchmark the Fuel Cost of Steam Generation
Benchmarking the fuel cost of steam generation, in dollars per 1,000 pounds $/1,000 lb) of steam, is an effective way to assess the efficiency of your steam system. This cost is dependent upon fuel type, unit fuel cost, boiler efficiency, feedwater temperature, and steam pressure. This calculation provides a good first approximation for the cost of generating steam and serves as a tracking device to allow for boiler performance monitoring. Table 1 shows the heat input required to produce 1 lb of saturated steam at different operating pressures and varying feed-water temperatures. Table 2 lists the typical energy content and boiler combustion efficiency for several common fuels.
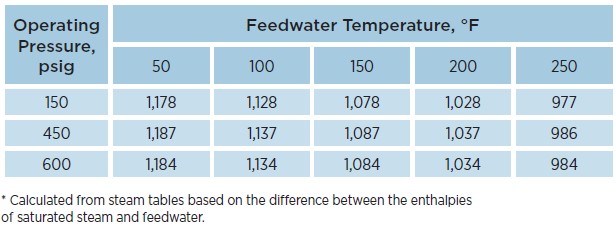

Data from the tables above can be used to determine the cost of usable heat from a boiler or other combustion unit. The calculations can also include the operat-ing costs of accessories such as feedwater pumps, fans, fuel heaters, steam for fuel atomizers and soot blowing, treatment chemicals, and environmental and maintenance costs.
Example
A boiler fired with natural gas costing $8.00/MMBtu produces 450-pounds-per-square-inch-gauge (psig) saturated steam and is supplied with 230°F feedwater. Using values from the tables, calculate the fuel cost of producing steam.
Effective Cost of Steam
The effective cost of steam depends on the path it follows from the boiler to the point of use. Take a systems approach and consider the entire boiler island, including effect of blowdown, parasitic steam consumption, and deaeration. Further complications arise because of the effects of process steam loads at different pressures, multiple boilers, and waste heat recovery systems. To determine the effective cost of steam, use a combined heat and power simulation model that includes all the significant effects.
Multi-Fuel Capability
For multi-fuel capability boilers, take advantage of the volatility in fuel prices by periodically analyzing the steam generation cost, and use the fuel that provides the lowest steam generation cost.
Higher Versus Lower Heating Values
Fuel is sold based on its gross or higher heating value (HHV). If, at the end of the combustion process, water remains in the form of vapor, the HHV must be reduced by the latent heat of vaporization of water. This reduced value is known as the lower heating value (LHV).
Minimize Boiler Short Cycling Losses
Boiler “short cycling” occurs when an oversized boiler quickly satisfies process or space heating demands, and then shuts down until heat is again required. Process heating demands can change over time. Boilers may have been oversized for additions or expansions that never occurred. Installing energy conservation or heat recovery measures may also reduce the heat demand. As a result, a facility may have multiple boilers, each rated at several times the maximum expected load.
Boilers used for space heating loads are often oversized, with their capacity chosen to meet total building heat losses plus heating of ventilation and infiltration air under extreme or design-basis temperature conditions. No credit is taken for thermal contributions from lights, equipment, or people. Excess capacity is also added to bring a facility to required settings quickly after a night setback.
Cycling Losses
A boiler cycle consists of a firing interval, a post-purge, an idle period, a pre-purge, and a return to firing. Boiler efficiency is the useful heat provided by the boiler divided by the energy input (useful heat plus losses) over the cycle duration. This efficiency decreases when short cycling occurs or when multiple boilers are operated at low firing rates.
This decrease in efficiency occurs, in part, because fixed losses are magnified under lightly loaded conditions. For example, if the radiation loss from the boiler enclosure is 1% of the total heat input at full-load, at half-load the losses increase to 2%, while at one-quarter load the loss is 4%. In addition to radiation losses, pre- and post-purge losses occur. In the pre-purge, the fan operates to force air through the boiler to flush out any combustible gas mixture that may have accumulated. The post-purge performs a similar function. During purging, heat is removed from the boiler as the purged air is heated.
Example
A 1,500 horsepower (hp) (1 hp = 33,475 Btu/hr) boiler with a cycle efficiency of 72.7% (E1) is replaced with a 600 hp boiler with a cycle efficiency of 78.8% (E2). Calculate the annual cost savings.
If the original boiler used 200,000 MMBtu of fuel annually, the savings from switching to the smaller boiler (given a fuel cost of $8.00/MMBtu) are:
Multiple Boiler Operations
The most efficient boilers should be brought on-line as loads increase, with less-efficient units taken off-line first as loads drop. Subject to emissions, operations, or firing rate limits, shift loads from a boiler where steam production is expensive to one where it is less expensive.
Use automatic controllers that determine the incremental costs (change in steam cost/change in load) for each boiler in the facility, and then shift loads accordingly. This maximizes efficiency and reduces energy costs. If possible, schedule loads to help optimize boiler system performance. Powerhouses containing multiple boilers that are simultaneously operated at low-fire conditions offer energy-saving opportunities for using proper boiler allocation strategies.
Boiler Downsizing
Fuel savings can be achieved by adding a smaller boiler sized to meet average loads at your facility, or by re-engineering the power plant to consist of multiple small boilers. Multiple small boilers offer reliability and flexibility to operators to follow load swings without over-firing and short cycling. Facilities with large seasonal variations in steam use operate small boilers when demand drops rather than operating their large boilers year-round.
Install Removable Insulation on Valves and Fittings
During maintenance, the insulation that covers pipes, valves, and fittings is often dam-aged or removed and not replaced. Pipes, valves, and fittings that are not insulated can be safety hazards and sources of heat loss. Removable and reusable insulating pads are available to cover almost any surface. The pads are made of a noncombustible inside cover, insulation material, and a noncombustible outside cover that resists tears and abrasion. Material used in the pads resists oil and water and has been designed for temperatures up to 1,600°F. Wire laced through grommets or straps with buckles hold the pads in place.
Applications
Reusable insulating pads are commonly used in industrial facilities for insulating flanges, valves, expansion joints, heat exchangers, pumps, turbines, tanks, and other irregular surfaces. The pads are flexible and vibration-resistant and can be used with equipment that is horizontally or vertically mounted or that is difficult to access. Any high-temperature piping or equipment should be insulated to reduce heat loss, reduce emissions, and improve safety. As a general rule, any surface that reaches temperatures greater than 120°F should be insulated to protect personnel. Insulating pads can be easily removed for periodic inspection or maintenance, and replaced as needed. Insulating pads can also contain built-in acoustical barriers to help control noise.
Energy Savings
The table below summarizes energy savings due to the use of insulating valve covers for a range of valve sizes and operating temperatures. These values were calculated using a computer program that meets the requirements of ASTM C 680—Heat Loss and Surface Temperature Calculations. Energy savings is defined as the difference in heat loss between the uninsulated valve and the insulated valve operating at the same temperature.
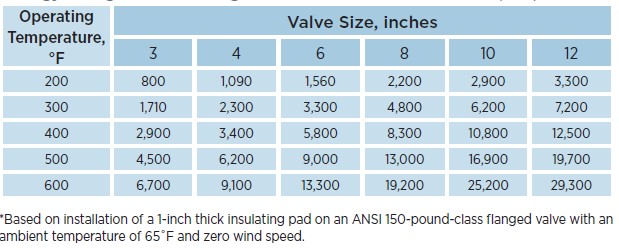
Example
Interpolating from the table above, calculate the annual fuel and dollar savings from installing a 1-inch thick insulating pad on an uninsulated 6-inch gate valve in a 250-pound-per-square-inch-gauge (psig) saturated steam line (406°F). Assume continuous operation with natural gas at a boiler efficiency of 80% and a fuel price of $8.00 per million Btu ($8.00/MMBtu).
Results:
Availability
Insulation supply companies are located regionally; this expedites delivery and helps meet site-specific job requirements. Most supply companies can take measurements on-site to ensure the best fit on irregular surfaces. For customized applications, manufacturers can provide instructions regarding the installation and removal of insulating pads.
Noise Control Benefits
Specify insulating pads that contain built-in barriers for noise control.
Insulation for Steam Traps
Effectively insulate inverted bucket traps with removable and reusable snap-on insulation. Thermostatic traps and disk traps should be insulated according to manufacturers’ specifications to ensure proper operation.
Before removal of all or any existing insulation material, check for asbestos in accordance with Occupational Safety and Health Administration (OSHA) regulations.
Steam Tip Sheet information adapted from information provided by the Industrial Energy Extension Service of Georgia Tech and reviewed by the AMO Steam Technical Subcommittee.
Deaerators in Industrial Steam Systems
Deaerators are mechanical devices that remove dissolved gases from boiler feedwater. Deaeration protects the steam system from the effects of corrosive gases. It accomplishes this by reducing the concentration of dissolved oxygen and carbon dioxide to a level where corrosion is minimized. A dissolved oxygen level of 5 parts per billion (ppb) or lower is needed to prevent corrosion in most high-pressure (>200 pounds per square inch) boilers. While oxygen concentrations of up to 43 ppb may be tolerated in low-pressure boilers, equipment life is extended at little or no cost by limiting the oxygen concentration to 5 ppb. Dissolved carbon dioxide is essentially completely removed by the deaerator.
How They Work
The design of an effective deaeration system depends upon the amount of gases to be removed and the final oxygen gas concentration desired. This in turn depends upon the ratio of boiler feedwater makeup to returned condensate and the operating pressure of the deaerator.
Deaerators use steam to heat the water to the full saturation temperature corresponding to the steam pressure in the deaerator and to scrub out and carry away dissolved gases. Steam flow may be parallel, cross, or counter to the water flow. The deaerator consists of a deaeration section, a storage tank, and a vent. In the deaeration section, steam bubbles through the water, both heating and agitating it. Steam is cooled by incoming water and condensed at the vent condenser. Noncondensable gases and some steam are released through the vent.
Steam provided to the deaerator provides physical stripping action and heats the mixture of returned condensate and boiler feedwater makeup to saturation temperature. Most of the steam will condense, but a small fraction (usually 5% to 14%) must be vented to accommodate the stripping requirements. Normal design practice is to calculate the steam required for heating and then make sure that the flow is sufficient for stripping as well. If the condensate return rate is high (>80%) and the condensate pressure is high in comparison to the deaerator pressure, then very little steam is needed for heating and provisions may be made for condensing the surplus flash steam.
Deaerator Steam Consumption
The deaerator steam consumption is equal to the steam required to heat incoming water to its saturation temperature, plus the amount vented with the non-condensable gases, less any flashed steam from hot condensate or steam losses through failed traps. The heat balance calculation is made with the incoming water at its lowest expected temperature. The vent rate is a function of deaerator type, size (rated feedwater capacity), and the amount of makeup water. The operating vent rate is at its maximum with the introduction of cold, oxygen-rich makeup water.
Additional Benefits
Deaerators provide the water storage capacity and the net positive suction head necessary at the boiler feed pump inlet. Returned condensate is mixed with makeup water within the deaerator. Operating temperatures range from 215° to more than 350°F, which reduces the thermal shock on downstream preheating equipment and the boiler.
Insulation
The deaerator section and storage tank and all piping conveying hot water or steam should be adequately insulated to prevent the condensation of steam and loss of heat.
Function Clarification
The deaerator is designed to remove oxygen that is dissolved in the entering water, not entrained air. Sources of “free air” include loose piping connections on the suction side of pumps and improper pump packing.
Pressure Fluctuations
Sudden increases in free or “flash” steam can cause a spike in deaerator vessel pressure, resulting in re-oxygenation of the feedwater. A dedicated pressure-regulating valve should be provided to maintain the deaerator at
a constant pressure.
Steam System Tip Sheets (18-20)
Cover Heated, Open Vessels
Open vessels that contain heated liquids often have high heat loss due to surface evaporation. Both energy and liquid losses are reduced by covering open vessels with insulated lids. The table below provides an estimate of the evaporative heat loss per square foot (ft2) of uncovered vessel surface area for various water and dry ambient air temperatures. It is assumed that the ambient air is dry with no wind currents. A fan pulling air over the uncovered tank could more than double the heat losses.
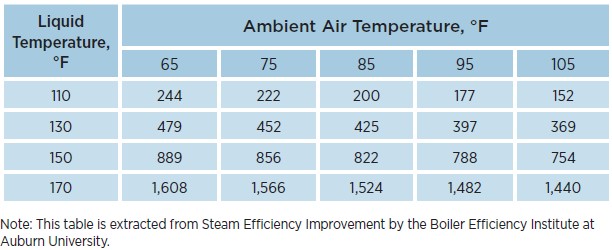
Example
A rinse tank is 4 feet (ft) wide and 10 feet (ft) long. It is maintained at a constant temperature of 170°F. Determine the evaporative heat loss from the tank if the ambient temperature is 75°F.
Cover the Tank with an Insulated Top
Assume that the rinse tank is heated during two shifts per day, five days per week, and 50 weeks per year. What are the annual energy savings that may be obtained by covering the tank? What is the heating cost reduction in a plant where the cost of steam is $8.00 per million Btu ($8.00/MMBtu)? Assume that covering the rinse tank with an insulated lid effectively reduces the heat losses from the liquid surface to a negligible value.
Heat Loss Detail
- Eliminating internal heat gains will also result in electrical energy savings if the open tanks are located within a conditioned space.
- Heat losses are a strong function of both wind velocity and ambient air humidity. A wind velocity of 3 miles per hour will more than double the rate of heat loss from a tank.
- Radiation heat transfer is a secondary source of tank surface heat losses. Radiation losses increase from 90 Btu/hr-ft2 at a liquid temperature of 110°F to 290 Btu/hr-ft2 at 190°F.
Replace Pressure-Reducing Valves with Backpressure Turbogenerators
Many industrial facilities produce steam at a pressure higher than that demanded by process requirements. Steam passes through pressure-reducing valves (PRVs, also known as letdown valves) at various locations in the steam distribution system to
let down or reduce its pressure. A noncondensing or backpressure steam turbine can perform the same pressure-reducing function as a PRV while converting steam energy into electrical energy.
In a backpressure steam turbogenerator, shaft power is produced when a nozzle directs jets of high-pressure steam against the blades of the turbine’s rotor. The rotor is attached to a shaft that is coupled to an electrical generator. The steam turbine does not consume steam. It simply reduces the pressure of the steam that is subsequently exhausted into the process header.
Cost-Effective Power Generation
In a conventional, power-only steam turbine installation, designers increase efficiency by maximizing the pressure drop across the turbine. Modern Rankine-cycle power plants with 1,800-pounds-per-square-inch-gauge (psig) superheated steam boilers and condensing turbines exhausting at near-vacuum pressures can generate electricity with efficiencies of approximately 40%.
Most steam users do not have the benefit of ultra-high-pressure boilers and cannot achieve such high levels of generation efficiency. However, by replacing a PRV with a backpressure steam turbine, where the exhaust steam is provided to a plant process, energy in the inlet steam can be effectively removed and converted into electricity. This means the exhaust steam has a lower temperature than it would have had if its pressure had been reduced through a PRV. In order to make up for this heat loss, steam plants with backpressure turbine installations increase their boiler steam throughput.
Thermodynamically, the steam turbine still behaves the same way that it would in a conventional Rankine-cycle power plant, achieving isentropic efficiencies of 20% to 70%. Economically, however, the turbine generates power at the efficiency of your particular steam boiler (modern steam boilers operate at approximately 80% efficiency), which then must be replaced with equivalent kilowatt-hours (kWh) of heat for downstream purposes. The resulting power generation efficiencies are well in excess of the average U.S. electricity grid generating efficiency of 33%. Greater efficiency means less fuel consumption; backpressure turbines can produce power at costs that are often less than $0.04/kWh.
Applicability
Packaged or “off-the-shelf” backpressure turbogenerators are now available in ratings as low as 50 kW. Backpressure turbogenerators should be considered when a PRV has constant steam flows of at least 3,000 pounds per hour (lb/hr), and when the steam pressure drop is at least 100 psi. The backpressure turbine is generally installed in parallel with the PRV.
Estimating Your Savings
To make a preliminary estimate of the cost of producing electrical energy from a backpressure steam turbine, divide your boiler fuel cost in dollars per million Btu ($8.00/MMBtu) by the product of your boiler efficiency (Eb, 80%) and electrical genera-tor efficiency (Eg, 95%). Then convert the resulting number into cost per kWh, as shown in the sample calculation below:
Electricity Cost = Fuel Cost ($/MMBtu) x 0.003412 MMBtu/kWh/(Eb x Eg)
Example: ($8.00/MMBtu x 0.003412 MMBtu/kWh)/(0.80 x 0.95) = $0.036/kWh
To estimate the potential power output at a PRV, refer to the figure below, which shows lines of constant power output, expressed in kW of electrical output per 1,000 pounds per hour (Mlb-hr) of steam throughput, as a function of turbine inlet and exhaust pressures. Look up your input and output pressure on the horizontal and vertical axes, and then use the reference lines to estimate the backpressure turbogenerator power output per Mlb-hr of steam flow. Then estimate the total installed generating capacity (kW) by multiplying this number by your known steam flow rate. The annual cost savings from the backpressure turbine can then be estimated as:
Power Output (kW) x Steam Duty (hr/yr) x (Cost of Grid Power
– Cost of Generated Power, $/kWh)
Life and Cost of Backpressure Turbogenerators
Turbogenerators with electrical switchgear cost about $900/kW for a 150 kW system to less than $200/kW for a 2,000 kW system. Installation costs vary, but typically average 75% of equipment costs.
Backpressure steam turbines are designed for a 20-year minimum service life and are known for having low maintenance requirements.
Consider Steam Turbine Drives for Rotating Equipment
Steam turbines are well suited as prime movers for driving boiler feedwater pumps, forced or induced-draft fans, blowers, air compressors, and other rotating equipment. This service generally calls for a backpressure noncondensing steam turbine. The low-pressure steam turbine exhaust is available for feedwater heating, preheating of deaerator makeup water, and/or process requirements.
Steam turbine drives are equipped with throttling valves or nozzle governors to modulate steam flow and achieve variable speed operation. The steam turbine drive is thus capable of serving the same function as an induction motor coupled to an inverter or adjustable speed drive. Steam turbine drives can operate over a broad speed range and do not fail when overloaded. They also exhibit the high starting torque required for constant torque loads such as positive displacement pumps.
Steam turbines are inherently rugged and reliable low-maintenance devices. They are easy to control and offer enclosed, nonsparking operation suitable for use in explosive atmospheres or highly corrosive environments. Steam turbines provide fast, reliable starting capability and are particularly adaptable for direct connection to equipment that rotates at high speeds. Steam turbine drives may be installed for continuous duty under severe operating conditions, or used for load shaping (e.g., demand limiting), standby, or emergency service.
Steam turbine performance is expressed in terms of isentropic efficiency or steam rate (the steam requirement of the turbine per unit of shaft power produced). Steam rates are given in terms of pounds per horsepower-hour (lb/hp-hr) or pounds per kilowatt-hour (lb/kWh).
Example
A 300-hp steam turbine has an isentropic efficiency of 43% and a steam rate of 26 lb/hp-hr given the introduction of 600-pounds-per-square-inch-gauge (psig)/750°F steam with a 40-psig/486°F exhaust. What steam flow is necessary to replace a fully-loaded 300-hp feedwater pump drive motor?
An examination of the ASME steam tables reveals that this steam turbine would utilize 103 Btu/lb of steam or 0.80 million Btu (MMBtu) of thermal energy per hour. Given a natural gas cost of $8.00/MMBtu and a boiler efficiency of 80%, the fuel-related cost of steam turbine operation is (0.80 MMBtu/hr/0.80 x $8.00/MMBtu) = $8.00/hr.
In comparison, a 300-hp motor with a full-load efficiency of 95% would require:
In this example, the steam turbine drive would provide energy cost savings when the price of electricity exceeds:
The total annual energy savings are strongly dependent upon the facility energy cost and the hours per year of feedwater pump operation. Annual energy savings are given in the table below for various electrical rates and pump operating schedules. In addition to operating cost savings, steam turbine maintenance costs should be compared with electric motor maintenance expenses.
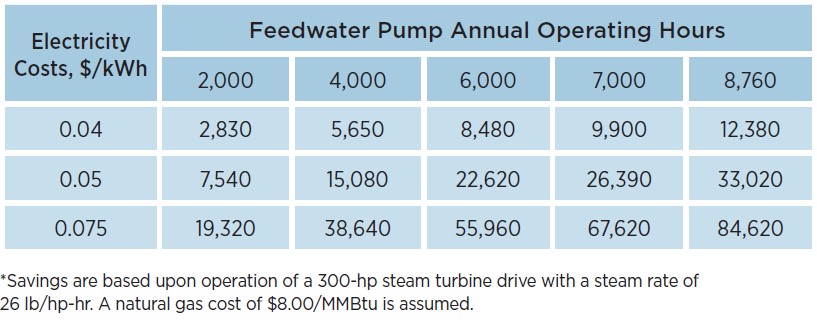
Steam Turbine Flexibility
Equipment redundancy and improved reliability can be obtained by mounting a steam turbine drive and an electric motor on opposite ends of the driven-equipment shaft. You can then select either the motor or turbine as the prime mover by increasing or decreasing the turbine speed relative to the synchronous speed of the motor.
Steam Tip Sheet information adapted from material provided by the TurboSteam Corporation and reviewed by the AMO Steam Technical Subcommittee.
Consider Installing High-Pressure Boilers with Backpressure Turbine-Generators
When specifying a new boiler, consider a high-pressure boiler with a backpressure steam turbine-generator placed between the boiler and the steam distribution network. A turbine-generator can often produce enough electricity to justify the capital cost of purchasing the higher-pressure boiler and the turbine-generator.
Since boiler fuel usage per unit of steam production increases with boiler pressure, facilities often install boilers that produce steam at the lowest pressure consistent with end use and distribution requirements.
In the backpressure turbine configuration, the turbine does not consume steam. Instead, it simply reduces the pressure and energy content of steam that is subsequently exhausted into the process header. In essence, the turbogenerator serves the same steam function as a pressure-reducing valve (PRV)—it reduces steam pressure—but uses the pressure drop to produce highly valued electricity in addition to the low-pressure steam. Shaft power is produced when a nozzle directs jets of high-pressure steam against the blades of the turbine’s rotor. The rotor is attached to a shaft that is coupled to an electrical generator.
Background
The capital cost of a back-pressure turbogenerator complete with electrical switchgear varies from about $900 per kilowatt (kW) for a small system (150 kW) to less than $200/kW for a larger system (>2,000 kW). Installation costs vary, depending upon pip-ing and wiring runs, but they typically average 75% of equipment costs.
Packaged or “off-the-shelf” backpressure turbogenerators are now available in ratings as low as 50 kW. Backpressure turbogenerators should be considered when a boiler has steam flows of at least 3,000 pounds per hour (lb/hr), and when the steam pressure drop between the boiler and the distribution network is at least 100 pounds per square inch gauge (psig). The backpressure turbine is generally installed in parallel with a PRV, to ensure that periodic turbine-generator maintenance does not interfere with plant thermal deliveries.
Cost-Effective Power Generation
In a backpressure steam turbine, energy from high-pressure inlet steam is efficiently converted into electricity, and low-pressure exhaust steam is provided to a plant process. The turbine exhaust steam has a lower temperature than the superheated steam created when pressure is reduced through a PRV. In order to make up for this heat or enthalpy loss and meet process energy requirements, steam plants with backpressure turbine installations must increase their boiler steam throughput (typically by 5% to 7%). Every Btu that is recovered as high-value electricity is replaced with an equivalent Btu of heat for downstream processes.
Thermodynamically, steam turbines achieve an isentropic efficiency of 20% to 70%. Economically, however, the turbine generates power at the efficiency of the steam boiler. The resulting power generation efficiency (modern steam boilers operate at approximately 80% efficiency) is well in excess of the efficiency for state-of-the-art single- or combined-cycle gas turbines. High efficiency means low electricity generat-ing costs. Backpressure turbines can produce electrical energy at costs that are often less than $0.04/kWh. The electricity savings alone—not to mention ancillary benefits from enhanced on-site electricity reliability and reduced emissions of carbon dioxide and criteria pollutants—are often sufficient to completely recover the cost of the initial capital outlay.
Estimating Your Savings
Since you have already determined that you need a boiler to satisfy your process thermal loads, the marginal cost of power produced from the backpressure turbine-generator is:
Cost of Power Production = (Annual Boiler Fuel Cost after Pressure Increase – Annual Boiler Fuel Cost before Pressure Increase)/Annual kWh Produced by Turbine-Generator
The cost of boiler fuel before and after a proposed pressure increase can be calculated directly from the boiler fuel cost, boiler efficiency, and inlet and outlet steam conditions. The annual kWh produced by the turbine generator can be calculated from the inlet and exhaust pressures at the turbine, along with the steam flow rate through the turbine, in thousand pounds per hour (Mlb-hr).
To estimate the potential power output of your system, refer to the figure below, which shows lines of constant power output, expressed in kW of electricity output per Mlb-hr of steam throughput as a function of the inlet and exhaust pressure through the turbine. Look up your input and output pressure on the axes shown, and then use the lines provided to estimate the power output, per Mlb/hr of steam flow rate for a backpressure turbogenerator. You can then estimate the turbine power output by multiplying this number by your known steam flow rate.
Example
A chemical company currently uses a 100-psig boiler with 78% boiler efficiency (E1) to produce 50,000 lb/hr of saturated steam for process loads. The boiler oper-ates at rated capacity for 6,000 hours per year (hr/yr). The boiler has reached the end of its service life, and the company is considering replacing the boiler with a new 100-psig boiler or with a high-pres-sure 600-psig boiler and a backpressure steam turbine-generator. Both new boiler alternatives have rated efficiencies (E2) of 80%. The company currently pays $0.06/kWh for electricity, and purchases boiler fuel for $8.00 per million Btu (MMBtu). Condensate return mixed with makeup water has an enthalpy of 150 Btu/lb. What are the relative financial merits of the two systems?
Step 1: Calculate the current annual boiler fuel cost: $3,200,000 per year
Current Boiler Fuel Cost
= Fuel Price x Steam Rate x Annual Operation x Steam Enthalpy Gain / E1
= $8.00/MMBtu x 50,000 lb/hr x 6,000 hr/yr x (1,190 Btu/lb – 150 Btu/lb) / (0.78 x 106 Btu/MMBtu)
= $3,200,000 per year
Step 2: Calculate the boiler fuel cost of a new 100-psig, low-pressure (LP) boiler: $3,120,000 per year
Resulting reductions in fuel costs are due solely to the higher efficiency of the new boiler.
New LP Boiler Fuel Cost
= Fuel Price x Steam Rate x Annual Operation x Steam Enthalpy Gain/E2
= $8.00/MMBtu x 50,000 lb/hr x 6,000 hr/yr x (1,190 Btu/lb – 150 Btu/lb)/ (0.80 x 106 Btu/MMBtu)
= $3,120,000 per year
Step 3: Calculate the boiler fuel cost of a new high-pressure (HP) boiler capable of producing 600 psig, 750ºF super-heated steam: $3,318,300 per year
We must now take into account the additional enthalpy necessary to raise the pressure of the boiler steam to 600 psig. With a 50% isentropic turbine efficiency, the exhaust steam from the backpressure turbine is at 100 psig and 527ºF and must be desuperheated by adding 5,000 lb/hr of water. In order to provide an equivalent amount of thermal energy to the process loads, the boiler steam output is reduced to 45,000 lb/hr.
New HP Boiler Fuel Cost
= Fuel Price x Steam Rate x Annual Operation x Steam Enthalpy Gain / E2
= $8.00/MMBtu x 45,000 lb/hr x 6,000 hr/yr x (1,379 Btu/lb – 150 Btu/lb) / (0.80 x 106 Btu/MMBtu)
= $3,318,300 per year
Step 4: Estimate the electricity output of the steam turbine-generator: 6,750,000 kWh per year
At 600-psig inlet pressure with 750ºF superheated steam and 100-psig exhaust pressure, the system will satisfy exist-ing steam loads but will also produce approximately 25 kW of electric power per Mlb-hr of steam production (you can use the figure on page 2 to estimate your power output for steam at saturated condi-tions). Thus,
Turbine-Generator Power Output
= 45 Mlb-hr x 25 kW/Mlb-hr
= 1,125 kW
Assuming a 6,000-hr operating year, the electricity output of this turbine will be: Turbine-Generator Electricity Output
= 1,125 kW x 6,000 hr/yr
= 6,750,000 kWh/yr
Step 5: Determine the cost of electricity produced by the turbine: $0.029/kWh
The value is derived from the difference in fuel costs between the two boiler alternatives, divided by the power produced by the turbine:
Fuel Cost of Produced Electricity
= ($3,318,300/yr – $3,120,000/yr)/ 6,750,000 kWh/yr
= $0.029/kWh
Step 6: Calculate energy savings benefits: $209,250 per year
Cost Savings = 6,750,000 kWh x ($0.06/kWh – $0.029/kWh) = $209,250/yr
This level of savings is often more than adequate to justify the capital and maintenance expenditures for the backpressure turbine-generator set and the incremental cost of purchasing and installing the higher-pressure boiler.
Steam System Tip Sheets (21-23)
Install an Automatic Blowdown-Control System
Background
To reduce the levels of suspended and total dissolved solids in a boiler, water is periodically discharged or blown down. High dissolved solids concentrations can lead to foaming and carryover of boiler water into the steam. This could lead to water hammer, which may damage piping, steam traps, or process equipment. Surface blowdown removes dissolved solids that accumulate near the boiler liquid surface and is often a continuous process.
Suspended and dissolved solids can also form sludge. Sludge must be removed because it reduces the heat-transfer capabilities of the boiler, resulting in poor fuel-to-steam efficiency and possible pressure vessel damage. Sludge is removed by mud or bottom blowdown.
During the surface blowdown process, a controlled amount of boiler water containing high dissolved solids concentrations is discharged into the sewer.
In addition to wasting water and chemicals, the blowdown process wastes heat energy, because the blowdown liquid is at the same temperature as the steam produced—approximately 366°F for 150-pounds-per-square-inch-gauge (psig) saturated steam—and blowdown heat recovery systems, if available, are not 100% efficient. (Waste heat may be recovered through the use of a blowdown heat exchanger or a flash tank in conjunction with a heat recovery system. For more information, see Steam Tip Sheet #10, Recover Heat from Boiler Blowdown.)
Advantages of Automatic Control Systems
With manual control of surface blowdown, there is no way to determine the concentration of dissolved solids in the boiler water, nor the optimal blowdown rate. Operators do not know when to blow down the boiler, or for how long. Likewise, using a fixed rate of blowdown does not take into account changes in makeup and feedwater conditions, or variations in steam demand or condensate return.
An automatic blowdown-control system optimizes surface-blowdown rates by regulating the volume of water discharged from the boiler in relation to the concentration of dissolved solids present. Automatic surface-blowdown control systems maintain water chemistry within acceptable limits, while minimizing blowdown and reducing energy losses. Cost savings come from the significant reduction in the consumption, disposal, treatment, and heating of water.

How it Works
With an automatic blowdown-control system, high- or low-pressure probes are used to measure conductivity. The conductivity probes provide feedback to a blowdown controller that com-pares the measured conductivity with a set-point value, and then transmits an output signal that drives a modulating blowdown release valve.
Conductivity is a measure of the electrical current carried by positive and negative ions when a voltage is applied across electrodes in a water sample. Conductivity increases when the dissolved ion concentrations increase.
The measured current is directly proportional to the specific conductivity of the fluid. Total dissolved solids, silica, chloride concentrations, and/ or alkalinity contribute to conductivity measurements. These chemical species are reliable indicators of salts and other contaminants in the boiler water.
Applications
Boilers without a blowdown heat-recovery system and with high blowdown rates offer the greatest energy-savings potential. The optimum blowdown rate is determined by a number of factors, including boiler type, operating pressure, water treatment, and makeup-water quality. Savings also depend upon the quantity of condensate returned to the boiler. With a low percentage of condensate return, more makeup water is required and additional blowdown must occur. Boiler blowdown rates often range from 1% to 8% of the feedwater flow rate, but they can be as high as 20% to maintain silica and alkalinity limits when the makeup water has a high solids content.
Price and Performance Example
For a 100,000 pound-per-hour (lb/hr) steam boiler, decreasing the required blowdown rate from 8% to 6% of the feedwater flow rate will reduce makeup water requirements by approximately 2,300 lb/hr. (See Steam Tip Sheet #9, Minimize Boiler Blowdown.) Annual energy, water, and chemicals savings due to blowdown rate reductions for a sample system are summarized in the table below. In many cases, these savings can provide a 1- to 3-year simple payback on the investment in an automatic blowdown- control system.
Purchasing and installing an automatic blowdown-control system can cost from $2,500 to $6,000. The complete system consists of a low- or high-pressure conductivity probe, temperature compensation and signal conditioning equipment, and a blowdown-modulating valve. Some systems are designed to monitor both feedwater and blowdown conductivity from multiple boilers. A continuous conductivity recording capability might also be desired. The total cost of the automatic blowdown system is dependent upon the system operating pressure and the design and performance options specified.
Recommended Practices
The American Society of Mechanical Engineers (ASME) has developed a consensus on operating practices for boiler blowdown. Sections VI and VII of the ASME Boiler and Pressure Vessel Code describe recommended practices. The ASME Boiler and Pressure Vessel Code can be ordered through the ASME website at www.asme.org.
Upgrade Boilers with Energy-Efficient Burners
Background
The purpose of the burner is to mix molecules of fuel with molecules of air. A boiler will run only as well as the burner performs. A poorly designed boiler with an efficient burner may perform better than a well-designed boiler with a poor burner. Burners are designed to maximize combustion efficiency while minimizing the release of emissions.
A power burner mechanically mixes fuel and combustion air and injects the mixture
into the combustion chamber. All power burners essentially provide complete combustion while maintaining flame stabilization over a range of firing rates. Different burners, however, require different amounts of excess air and have different turndown ratios. The turndown ratio is the maximum inlet fuel or firing rate divided by the minimum firing rate.
An efficient natural gas burner requires only 2% to 3% excess oxygen, or 10% to 15% excess air in the flue gas, to burn fuel without forming excessive carbon monoxide. Most gas burners exhibit turndown ratios of 10:1 or 12:1 with little or no loss in combustion efficiency. Some burners offer turndowns of 20:1 on oil and up to 35:1 on gas. A higher turndown ratio reduces burner starts, provides better load control, saves wear and tear on the burner, reduces refractory wear, reduces purge-air requirements, and provides fuel savings.
Efficient Burner Technologies
An efficient burner provides the proper air-to-fuel mixture throughout the full range of firing rates, without constant adjustment. Many burners with complex linkage designs do not hold their air-to-fuel settings over time. Often, they are adjusted to provide high levels of excess air to compensate for inconsistencies in the burner performance.
An alternative to complex linkage designs, modern burners are increasingly using servomotors with parallel positioning to independently control the quantities of fuel and air delivered to the burner head. Controls without linkage allow for easy tune-ups and minor adjustments, while eliminating hysteresis, or lack of retraceability, and provide accurate point-to-point control. These controls provide consistent performance and repeatability as the burner adjusts to different firing rates.
Alternatives to electronic controls are burners with a single drive or jackshaft. Avoid purchasing standard burners that make use of linkages to provide single-point or proportional control. Linkage joints wear and rod-set screws can loosen, allowing slip-page, the provision of suboptimal air-to-fuel ratios, and efficiency declines.
Applications
Consider purchasing a new energy-efficient burner if your existing burner is cycling on and off rapidly. Rotary-cup oil burners that have been converted to use natural gas are often inefficient. Determining the potential energy saved by replacing your existing burner with an energy-efficient burner requires several steps. First, complete recommended burner-maintenance requirements and tune your boiler. Conduct combustion-efficiency tests at full- and part-load firing rates. Then, compare the measured efficiency values with the performance of the new burner. Most manufacturers will provide guaranteed excess levels of oxygen, carbon monoxide, and nitrous oxide.
Example
Even a small improvement in burner efficiency can provide significant savings. Consider a 50,000 pound-per-hour process boiler with a combustion efficiency of 79% (E1). The boiler annually consumes 500,000 million Btu (MMBtu) of natural gas. At a price of $8.00/MMBtu, the annual fuel cost is $4 million. What are the savings from an energy-efficient burner that improves combustion efficiency by 1%, 2%, or 3%?
Cost Savings = Fuel Consumption x Fuel Price x (1 – E1/E2)
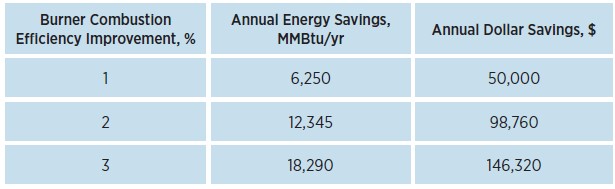
If the installed cost is $75,000 for a new burner that provides an efficiency improvement of 2%, the simple payback on investment is:
Simple Payback = $75,000/$98,760/year = 0.76 year
Maintenance Requirements
Conduct burner maintenance at regular intervals. Wear on the firing head, diffuser, or igniter can result in air leakage or failure of the boiler to start. One burner distributor recommends maintenance four times per year, with the change of seasons. A change in weather results in a change in combustion.
Fan Selection
Fan selection is also important. Backward-curved fans provide more reliable air control than forward-curved fans. Radial-damper designs tend to provide more repeatable air control at lower firing rates than blade-type damper assemblies.
Steam Tip Information is adapted from material supplied by PBBS Equipment Corp. and Blesi-Evans Company and reviewed by the AMO Steam Technical Subcommittee. For additional information, refer to Steam Tip Sheet #4, Improve Your Boiler’s Combustion Efficiency.
Consider Installing Turbulators on Two- and Three-Pass Firetube Boilers
Firetube Boilers
The packaged firetube boiler is the most common boiler design used to provide heating or process steam in industrial and heavy commercial applications. The American
Boiler Manufacturers Association (ABMA) surveyed sales of high-pressure [15- to 350-pounds-per-square-inch-gauge (psig)] firetube and small watertube boilers between 1978 and 1994. ABMA found that firetube boilers comprised more than 85% of the sales of these boilers to industry.
Although firetube boilers are available in ratings up to 85,000 pounds of steam per hour (lb/hr), they are generally specified when the required steam pressure is under 150 psig and the boiler capacity is less than 25,000 lb/hr. Watertube boilers are designed for larger, high-pressure, and superheated steam applications.
In a firetube boiler, hot combustion gases pass through long, small-diameter tubes, where heat is transferred to water through the tube walls. Firetube boilers are categorized by their number of “passes,” or the number of times that the hot combustion gases travel across the boiler heat-exchange surfaces. For example, a two-pass boiler provides two opportunities for hot gases to transfer heat to the boiler water. Hot combustion gases enter the tubes in a turbulent flow regime, but within a few feet, laminar flow begins and a boundary layer of cooler gas forms along the tube walls. This layer serves as a barrier, retarding heat transfer.
Turbulators, which consist of small baffles, angular metal strips, spiral blades, or coiled wire, are inserted into the boiler tubes to break up the laminar boundary layer. This increases the turbulence of the hot combustion gases and the convective heat transfer
to the tube surface. The result is improved boiler efficiency. Turbulators are usually installed on the last boiler pass.
Turbulator installers can also balance gas flow through the tubes by placing longer turbulators in the uppermost tubes. This practice increases the effectiveness of the available heat-transfer surface by eliminating thermal stratification and balancing the gas flow through the firetubes.
Applications
Turbulators can be a cost-effective way to reduce the stack temperature and increase the fuel-to-steam efficiency of single-pass horizontal return tubular (HRT) brick-set boilers and older two- and three-pass oil- and natural-gas-fueled firetube boilers. Turbulators are not recommended for four-pass boilers or coal-fired units. A four-pass unit provides four opportunities for heat transfer. It has more heat exchange surface area, a lower stack temperature, higher fuel-to-steam efficiency, and lower annual fuel costs than a two- or three-pass boiler operating under identical conditions. New firetube boilers perform better than older two- and three-pass designs.
Turbulators can also be installed to compensate for efficiency losses when a four-pass boiler is being converted to a two-pass boiler because of door warpage and loose and leaking tubes.
Turbulators are substitutes for more costly economizers or air-preheaters. They are simple, easy to install, and low cost. Their installed cost is about $10 to $15 per boiler tube. Current turbulator designs do not cause a significant increase in pressure drop or contribute to soot formation in natural-gas-fired boilers. Turbulators are held in place with a spring lock and are easily removed to allow for tube brushing.
Turbulators come in various lengths and widths and should be installed by a qualified installer. To avoid combustion problems, the boiler burner should be retuned after the turbulators have been installed. The installer must also verify that the stack temperature does not fall below the flue gas dew point.
Price and Performance Example
A manufacturing facility installed 150 turbulators into its firetube boiler. Tests conducted both before and after turbulator installation indicated a reduction in the stack gas temperature of 130°F. More combustion heat was being transferred into the boiler water. Because each 40°F reduction in the boiler flue gas temperature results in a 1% boiler-efficiency improvement, overall boiler efficiency has improved by about 3.25%. Fuel costs have decreased by approximately 4%.
Example
Consider a two-pass firetube boiler that consumes 60,000 million Btu (MMBtu) of natural gas annually while producing 15,000 lb/hr of 100-psig saturated steam. What are the annual energy and cost savings, given that the installation of turbulators improves the boiler efficiency from 79% (E1) to 82% (E2)? Natural gas is priced at $8.00/MMBtu.
If the boiler has 250 tubes and the installed cost for the turbulator is $15 per tube, the simple payback on the investment in the energy efficiency measure is:
Steam System Tip Sheets (24-26)
Consider Installing a Condensing Economizer
The key to a successful waste heat recovery project is optimizing the use of the recovered energy. By installing a condensing economizer, companies can im-prove overall heat recovery and steam system efficiency by up to 10%. Many boiler applications can benefit from this additional heat recovery, such as district heating systems, wallboard production facilities, greenhouses, food processing plants, pulp and paper mills, textile plants, and hospitals. Condensing economizers require site-specific engineering and design, and a thorough understanding of the effect they will have on the existing steam system and water chemistry.
Use this tip sheet and its companion, Considerations When Selecting a Condensing Economizer, to learn about these efficiency improvements.
A conventional feedwater economizer reduces steam boiler fuel requirements by transferring heat from the flue gas to the boiler feedwater. For natural gas-fired boilers, the lowest temperature to which flue gas can be cooled is about 250°F to prevent condensation and possible stack or stack liner corrosion.
The condensing economizer improves waste heat recovery by cooling the flue gas below its dew point, which is about 135°F for products of combustion of natural gas. The economizer reclaims both sensible heat from the flue gas and latent heat by condensing flue gas water vapor (see Table 1). All hydrocarbon fu-els release significant quantities of water vapor as a combustion byproduct. The equation below shows the reactants and combustion products for the stoichio-metric combustion in air of methane (CH4), the primary constituent of natural gas. When one molecule of methane is burned, it produces two molecules of water vapor. When moles are converted to pound/mole, we find that every pound of methane fuel combusted produces 2.25 lb. of water vapor, which is about 12% of the total exhaust by weight.
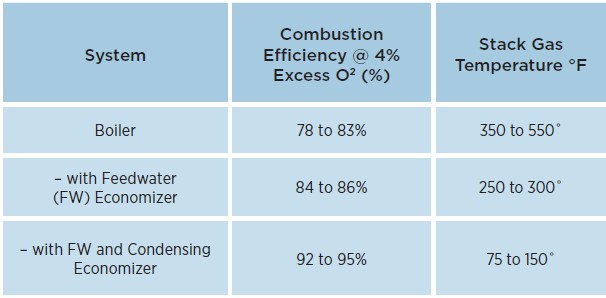
Since the higher heating value of methane is 23,861 Btu per pound (Btu/lb), 41.9 lb of methane is required to provide one million Btu (MMBtu) of energy, resulting in 94.3 lb of high temperature water vapor. The latent heat of vaporization of water under atmospheric pressure is 970.3 Btu/lb. When one MMBtu of methane is com-busted, 91,495 Btu of water vapor heat of evaporation (94.3 lb x 970.3 Btu/lb) is released up the boiler stack. This la-tent heat represents approximately 9% of the initial fuel energy content. The bulk of this latent heat can be recovered by cooling the exhaust gas below its dew point using a direct contact or indirect condensing economizer. It is possible to heat water to about 200°F with an indirect economizer or 140°F with a direct contact economizer.

Energy Savings Potential
The available heat in a boiler’s exhaust gases is dependent upon the hydrogen content of the fuel, the
fuel firing rate, the percent of excess oxygen in the flue gases, and the stack gas temperature.
Consider a natural gas-fired boiler that produces 100,000 lb/hr of 100-psig saturated steam. At 83% efficiency, the boiler firing rate is about 116 MMBtu/hr. At its full firing rate, the boiler consumes over 4,860 lb of natural gas each hour while exhausting 10,938 lb of high temperature water vapor each hour. The water vapor in the flue gas contains over 10.6 MMBtu/hr of latent heat. As shown in Table 2, the total heat actually available for recovery is strongly dependent upon the stack gas temperature at the condensing economizer outlet.
Assume that an indirect contact condensing economizer is retrofitted onto this 100,000 lb/hr steam boiler to heat 50% of the makeup water from 55°F to 200°F and flue gases are cooled to 100°F. At these conditions, 12.75 MMBtu/hr of total energy is available in the exhaust, of which 7.55 MMBtu/hr will be recovered to heat makeup water in the condensing economizer. More energy could be recovered if additional heat sinks are available. Given 8,000 hours per year of boiler operation, and a fuel cost of $8.00/MMBtu, the annual energy recovered is valued at:
Annual Savings
= 7.55 MMBtu/hr x 8,000 hrs/ yr x $8.00/MMBtu/0.83
= $582,170
Examples
District Heating System
A boiler plant that provides up to 500,000 lb/hr of steam for a district heating system installed a direct contact condensing economizer. This economizer saves up to 20 MMBtu/hr, depending on the boiler load. Since condensate is not returned from the district heating system, the recovered energy is used to preheat plant makeup water from 45°- 60°F up to 132°F, resulting in a steam system energy efficiency improvement of 6.3%.
Food Processing Plant
A food processing plant installed an indirect contact condensing economizer on a 20,000-lb/hr boiler. The condensing economizer reduced the flue gas tempera-ture from 300°F to 120°F, while capturing 2.0 MMBtu/hr of sensible and latent heat. Energy recovered by the condensing economizer heated makeup water, reducing deaerator steam requirements from 5,000 lb/hr to 1,500 lb/hr.
Considerations When Selecting a Condensing Economizer
Boilers equipped with condensing economizers can have an overall efficiency that exceeds 90%. A condensing economizer can increase overall heat recovery and steam system efficiency by up to 10% by reducing the flue gas temperature below its dew point, resulting in improved effectiveness of waste heat recovery.
This tip sheet is a companion to Steam Tip Sheet 26A, Consider Installing a Condensing Economizer, and discusses two types of condensing economizer: indirect and direct contact.
An indirect contact condensing economizer (see Figure 1) removes heat from hot flue gases by passing them through one or more shell-and-tube or tubular heat exchangers. This economizer can heat fluids to a temperature of 200°F while achieving exit gas temperatures as low as 75°F. The indirect contact economizer is able to preheat water to a higher outlet or process supply tempera-ture than the direct contact economizer. The condensing economizer must be designed to withstand corrosion from condensed water vapor produced by the combustion of hydrocarbon fuels such as natural gas or light oils. The condensed water vapor is acidic and must be neutralized if it is to be discharged into the sewer system or used as process water.

Another heat recovery option is to use a direct contact condensing economizer (see Figure 2), which consists of a vapor-conditioning chamber followed by a countercurrent spray chamber. In the spray chamber, small droplets of cool liquid come into direct contact with the hot flue gas, providing a non-fouling heat transfer surface. The liquid droplets cool the stack gas, con-dense and disentrain the water vapor. The spray chamber may be equipped with packing to improve contact between the water spray and hot gas. A mist eliminator is required to prevent carryover of small droplets. The direct contact design offers high heat transfer coupled with water recovery capability since heated water can be collected for boiler feedwater, space heating, or plant process needs. Recovered water will be acidic and may require treatment prior to use, such as membrane technology, external heat exchangers, or pH control. Water filtration will also be required for fuel types other than natural gas.
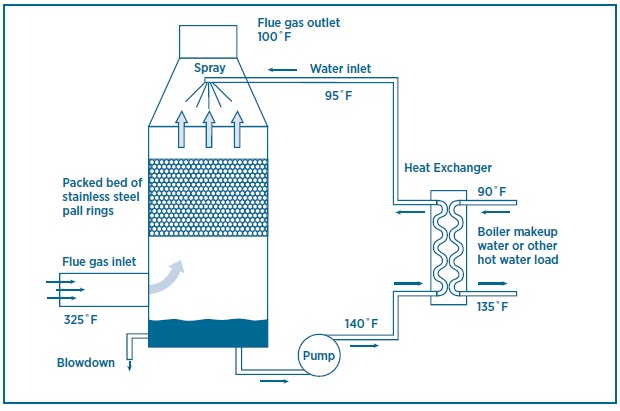
The site must have substantial heating requirements for low-temperature process or cold makeup water if a direct contact condensing economizer is to be a viable heat recovery alterna-tive. Because direct contact condensing economizers operate close to atmospher-ic pressure, altitude and flue gas tem-perature limit makeup water temperature to 110°F to 140°F.
When considering whether to install a condensing economizer, evaluate changes in system operating parame-ters. These economizers preheat boiler makeup water and reduce deaerator steam requirements, thereby providing more steam for plant processes. The energy savings potential is decreased if the majority of the deaerator steam is supplied from blowdown heat recovery. The condensing economizer could also limit or decrease backpressure steam turbine energy production if the turbine discharge is used to balance a low-pressure header. The reduction in stack gas exit temperature reduces plume buoyancy and must be considered when modeling pollutant dispersion. Performance characteristics of both indirect and direct contact economizers are summarized in the table below.
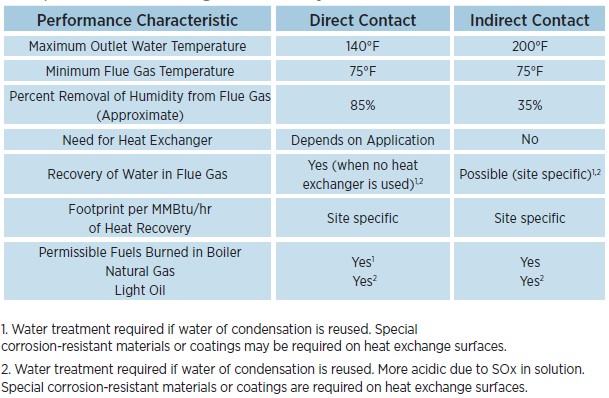
Condensing economizers require site-specific engineering and design, and a thorough understanding of the effect their operation will have on the existing steam system and water chemistry.
Use Steam Jet Ejectors or Thermocompressors to Reduce Venting of Low-Pressure Steam
Large industrial plants often vent significant quantities of low-pressure steam to the atmosphere, wasting energy, water, and water-treatment chemicals. Recovery of the latent heat content of low-pressure steam reduces the boiler load, resulting in energy and fuel cost savings. Low-pressure steam’s potential uses include driving evaporation and distillation processes, producing hot water, space heating, producing a vacuum, or chilling water. If the steam pressure is too low for the intended application, a steam jet thermocompressor can boost the pressure and temperature to the required level.
Operating Principles
Thermocompressors and ejectors operate on the same thermodynamic and physical principle: energy contained in high-pressure steam can be transferred to a lower pressure vapor or gas to produce a mixed discharge stream of intermediate pressure. These devices are known for:
- Simple construction
- Easy installation
- Easy maintenance with no moving parts
- Insensitivity to fouling
- Low capital and installation costs
- Long useful operating lives
If the objective is to recover the latent heat content of the low-pressure suction vapor for process use, the device is called a thermocompressor. If the objective is to pull a vacuum on a process vessel, the device is called an ejector.
Boosting Steam Pressure and Temperature with Thermocompressors
Single- or multi-stage thermocompressors are used to boost low-pressure vent steam to a useful higher pressure and temperature. When high-pressure motive steam is available, thermocompressors can be economically used for compression ratios up to 6:1 (absolute pressure of supply steam/suction steam).
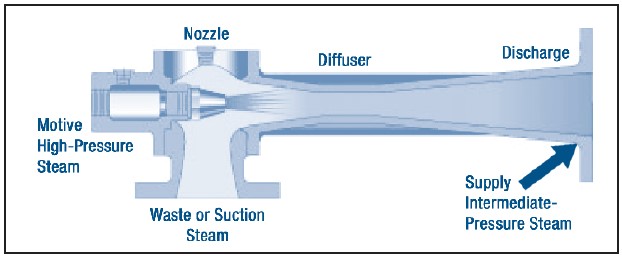
High-pressure motive steam supplied to the thermocompressor expands in a converging-diverging nozzle to convert pressure energy to kinetic energy. Vent steam supplied to the suction port is entrained into this low-pressure/high-velocity jet, where mixing occurs. The diffuser portion of the thermocompressor shown in the figure reconverts the kinetic energy of the mixture back into pressure. The intermediate discharge pressure is between the pressures of the motive and low-pressure suction steam. Discharge pressure is determined by the compression ratio (i.e., the ratio of the pounds per hour [lb/hr] of motive steam supplied to the lb/hr of low-pressure suction steam entrained). Thermocompressor capacity of the device is dictated by the availability of motive steam, motive and suction steam pressure, and discharge steam pressure requirements. Applications include drying and heating, multi-effect evaporators, vulcanizers, reboilers, strippers, condensate receiver tanks, and solvent extraction processes.
Producing a Vacuum with Steam Jet Ejectors
In a vacuum jet ejector, the low-pressure vent steam is the motive energy source. Air and/or water vapor are sucked from the process into the mixing chamber at near-vacuum conditions. Both the motive vent steam and the suction vapors/gases pass through a venturi throat and are released through a diffuser. Although steam jet ejectors can be used to pull a vacuum with motive steam pressure as low as 5 pounds per square inch gauge (psig), higher pressures of 15 to 50 psig are more practical.
Example
An industrial facility vents 10,000 lb/hr of steam at near atmospheric pressure [0.3 psig, 212.9°F, 1,150.7 Btu per pound (Btu/lb)]. Wasted steam can be converted into useful low-pressure process steam by boosting its pressure to 15.3 psig (250.3°F, 1,164.1 Btu/lb). Saturated motive steam at a pressure of 200 psig is available (387°F, 1,199.7 Btu/lb).
For a required compression ratio of 2:1 (15.3 + 14.7 psia)/(0.3 + 14.7 psia), 1.1 lb of high-pressure motive steam per lb of low-pressure suction steam is required. The thermo-compressor requires 11,000 lb/hr of 200-psig steam to produce a discharge of 21,000 lb/hr of 15.3-psig intermediate-pressure steam. Elimination of steam venting saves:
For a continuously operating facility, annual energy savings are approximately
94,000 MMBtu. For natural gas fuel priced at $8.00 per MMBtu ($8.00/MMBtu) with a boiler efficiency of 80%, fuel savings are valued at: